Synapses and Neurotransmitter Receptors
John Tuthill
Learning Objectives
1. Describe how neurotransmitter is released at chemical synapses, including the role of calcium.
The neurotransmitter at chemical synapses is packaged in intracellular vesicles. A presynaptic action potential causes presynaptic calcium to rise. Calcium is the trigger to release the contents of synaptic vesicles by the process of exocytosis.
2. Compare excitatory and inhibitory neurotransmitters and identify the major examples of each neurotransmitter type in the CNS.
The ‘workhorse’ neurotransmitters in the CNS are glutamate, which is excitatory and GABA and glycine, which are inhibitory. Outside the CNS, acetylcholine is excitatory.
3. Compare ionotropic and metabotropic receptors.
Ionotropic receptors are ion channels that open rapidly to change membrane potential. Metabotropic receptors are GPCRs that initiate second messenger signaling and hence produce slower onset and longer lasting changes in membrane potential.
4. Describe the mechanism by which neurotransmitter is cleared at chemical synapses.
Membrane transporters clear glutamate, GABA, and glycine from the extracellular space into glia or back into neurons. Acetylcholine is destroyed by the extracellular enzyme acetylcholinesterase.
5. Describe how temporal and spatial summation of synaptic potentials affect postsynaptic responses.
Postsynaptic electrical responses are augmented by summation when one presynaptic nerve terminal fires rapidly in succession or when several nearby nerve terminals fire nearly synchronously.
6. Outline the key differences between chemical and electrical synapses.
Overall Summary
Chemical synapses use neurotransmittters to activate postsynaptic receptors and deliver excitatory or inhibitory messages. The receptors amplify the message. In contrast, electrical synapses transmit by electric currents flowing through gap junctions between two cells without amplification and without the possibility of sign inversion.
Synapses are the communication junctions formed by neurons to each other and to the cells of their target tissues. The word synapse comes from Greek, meaning joining together, and the process of communication is called synaptic transmission. This is a fundamentally important property; a nervous system without synaptic transmission would be like a society without language or any other form of communication. In addition to transmitting signals from one cell to another, synapses are the likely sites for many key brain functions such as decision making, learning, and memory.
Chemical synapses are the predominant form of synaptic transmission in the brain. Transmission at these synapses is mediated by a diffusible chemical transmitter released by the presynaptic cell in response to a change in voltage. Electrical synapses, in contrast, work through the direct flow of ions and other small molecules from one cell to another. They do not involve a chemical transmitter. Electrical synaptic transmission is relatively uncommon in the brain, but such electrical communication is important in sensory systems (e.g. retina), the liver, and cardiac muscle among other places. Electrical transmission is particularly effective at synchronizing the electrical signals in collections of cells – e.g. causing muscle cells to contract at the same time.
(Unless otherwise noted, all figures are from: Kandel ER, Schwartz JH, Jessell TM 2012, Siegelbaum SA, Hudspeth AJ. ‘Principles of Neural Science’, 5th ed. McGraw-Hill, New York.)
Basic sequence of events
The mechanisms governing transmitter release were first revealed in studies of neuromuscular junction. The neuromuscular junction is the chemical synapse formed by a motoneuron innervating a skeletal muscle fiber (Figure 1). While this synapse has several unique features, it also exhibits many general features of chemical synapses, and indeed was the place where many of these general features were first discovered. The basic sequence of events at neuromuscular junctions is as follows (Figure 2):
1. A nerve action potential – a large, rapid and discrete change in voltage – propagates down the axon of the motoneuron and depolarizes its synaptic terminal.
2. The presynaptic depolarization due to the action potential causes voltage-gated Ca2+ channels to open and to permit Ca2+ to enter the synaptic terminal.
3. Ca2+ entry is the signal to trigger exocytosis of presynaptic vesicles, which in motoneurons are filled with acetylcholine (ACh).
4. Released ACh quickly diffuses across the narrow synaptic cleft to the skeletal muscle fiber where it binds to nicotinic ACh receptors on the muscle membrane. These receptors are non-selective cation channels having about equal permeability to K+ and Na+. Binding of ACh opens these ACh receptor channels, leading to a local depolarization of the muscle called the end-plate potential. Under normal circumstances, the large synaptic depolarization always reaches firing threshold and gives rise to a muscle membrane action potential that propagates in both directions in the muscle.
Each action potential causes the muscle fiber to contract. Diseases that target the neuromuscular junction, for example myasthenia gravis, can reduce the end-plate potential so it sometimes fails to elicit an action potential in the muscle. Such failures manifest as muscle weakness.
5. ACh is removed from the synaptic cleft both by enzymatic degradation (by acetylcholinesterase) and by diffusion.
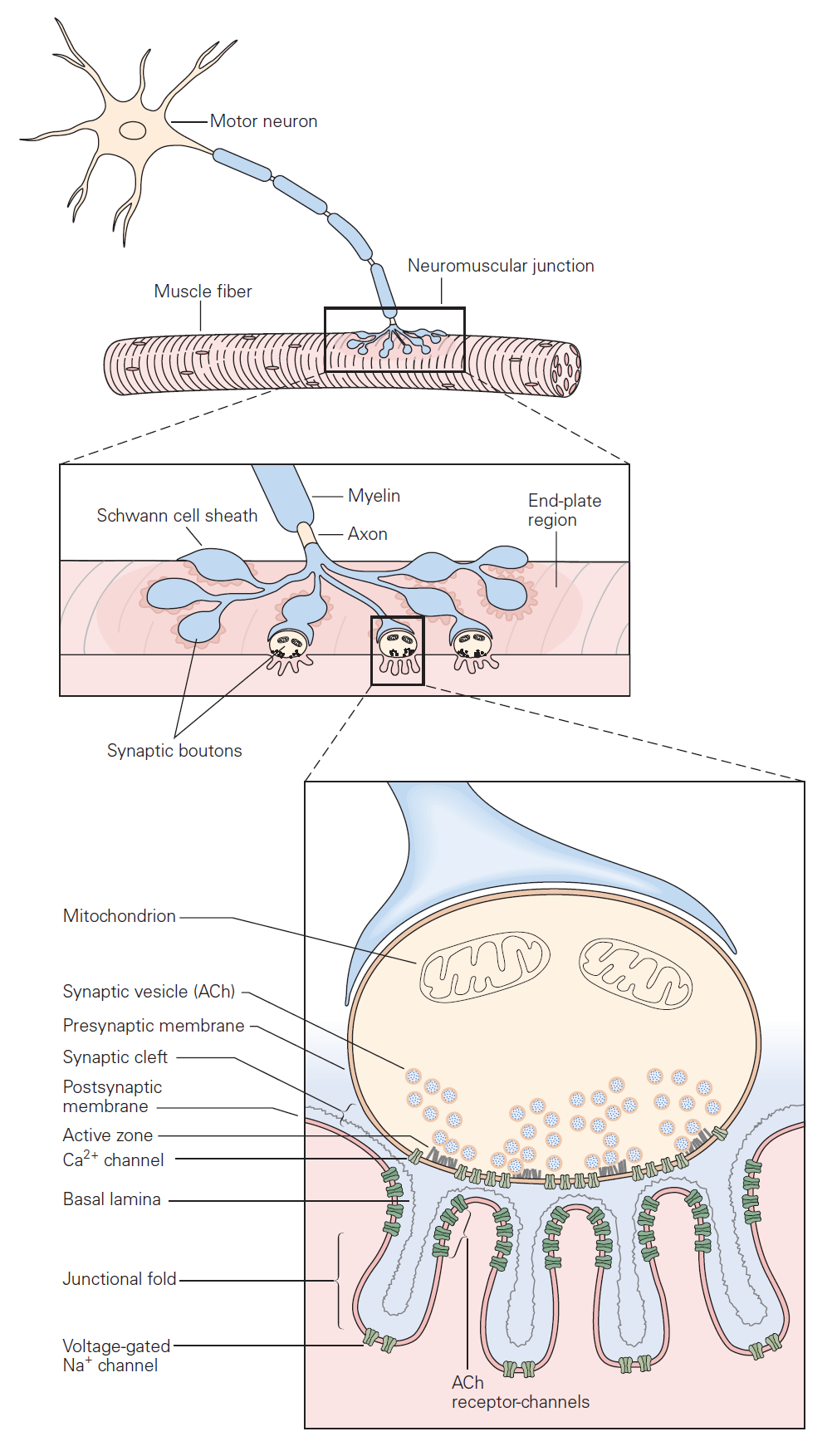
Neurotransmitter release
Classic studies at the neuromuscular junction by Bernard Katz and colleagues revealed two features of neurotransmitter release that are shared by all chemical synapses (Figure 2):
(1) The chemical neurotransmitter is stored in presynaptic vesicles.
(2) Intracellular Ca2+ is the trigger signal for neurotransmitter release.
When an action potential propagates into the synaptic terminal, the resulting depolarization leads to the opening of voltage-gated Ca2+ channels and Ca2+ entry into the synaptic terminal. Like voltage-gated Na+ and K+ channels that produce action potentials, these voltage-gated Ca2+ channels open in direct response to depolarization. They contrast with ligand-gated channels that open due to the binding of a chemical ligand (e.g. the ACh channels expressed on the muscle at neuromuscular junction). The influx of Ca2+ and the resulting increase in internal Ca2+ concentration acts on proteins that control exocytosis. You have heard about these SNARE proteins already (see the class on Cell biology of secretion); they are a key part of conserved machinery involved in the release of chemical neurotransmitters. Ca2+-induced conformational changes in these proteins lead to the fusion of synaptic vesicles with the presynaptic cell membrane; neurotransmitter stored in the vesicles can then diffuse across the synaptic cleft to act on receptors in the postsynaptic cell (see below). The increase in internal Ca2+ is a requisite signal to initiate neurotransmitter release.
These properties — storage of transmitter in vesicles and the requirement for Ca2+ in triggering vesicle fusion — are conserved across all chemical synapses. Further, the protein machinery involved in exocytosis is largely conserved across synapses and is the target of several toxins such as botulinum and tetanus toxins. Botulinum toxin causes flaccid muscle paralysis by cleaving SNARE proteins and hence rendering presynaptic motoneurons incapable of transmitter release. In cosmetic medicine, this toxin under the name Botox is used to relax wrinkles caused by contraction of facial muscles.
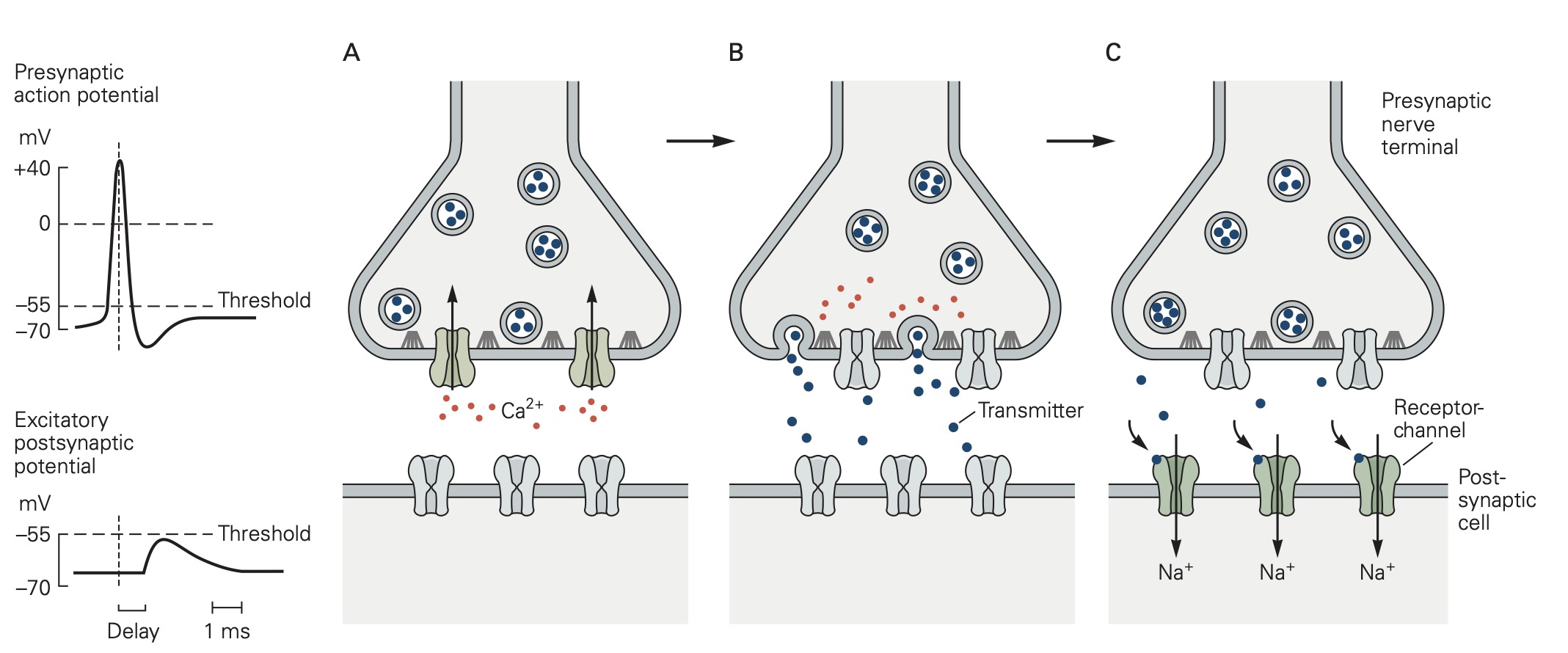
Diversity of central synapses
The neuromuscular junction is a highly specialized giant synapse — a one-to-one contact in which each action potential in the nerve is faithfully transmitted to the muscle under normal circumstances. Synapses between neurons in the nervous system show considerably more diversity. This diversity arises from differences in the identity of the released neurotransmitters, differences in the type of receptors that the neurotransmitters act on, and differences in how multiple synaptic inputs are combined or integrated by the postsynaptic cell. Below we consider each of these issues in more detail.
Postsynaptic receptors at central synapses
At all chemical synapses, as at the neuromuscular junction, released chemical transmitter diffuses across the synaptic cleft and binds to and activates receptors on the post-synaptic cell. Receptor activation leads to a conductance change (almost always a conductance increase, due to opening of ion channels), and this in turn leads to a synaptic potential in the postsynaptic cell. The synaptic potential is a local, graded change in membrane voltage analogous to the end-plate potential at neuromuscular junctions. In the CNS, the synaptic potential from a single excitatory nerve synapse is typically quite small and would not by itself initiate an action potential in the postsynaptic cell (see discussion of integration below). In this respect most synaptic potentials are quite different from single end-plate potentials, which in normal muscle always lead to an action potential. As described below, synaptic potentials in the CNS can be depolarizing (excitatory postsynaptic potentials or EPSPs) or hyperpolarizing (inhibitory postsynaptic potentials or IPSPs). Key types of receptors mediating these different synaptic potentials are summarized in the following table.
Synaptic potential | transmitter | Receptor type | Predominant location |
Fast EPSP | glutamate ACh | NMDA, kainate, AMPA Nicotinic ACh receptor | Central nervous system Skeletal muscle autonomic ganglia |
Fast IPSP | GABA
glycine |
GABAA receptor Glycine receptor | Central nervous system Spinal cord |
Slow EPSP | ACh | Muscarinic ACh | Smooth muscle |
Slow IPSP | GABA
ACh |
GABAB receptor Muscarinic ACh receptor | Central nervous system
heart |
Table 1: Neurotransmitters mediating several key types of synaptic potentials. Fast synaptic potentials are mediated by ionotropic receptors and slow synaptic potentials by GPCR metabotropic receptors (see Learning Objective #3).
Synaptic potentials can be depolarizing (excitatory) or hyperpolarizing (inhibitory). The main ions involved in the generation of different synaptic potentials are Na+, K+ and Cl–. Their equilibrium potentials are approximately ECl ~ -90 mV, EK ~ -100 mV, and ENa ~ +70 mV. These values should be familiar! The rules for how changes in the permeability of the membrane to these ions affects the membrane potential are the same as those you learned in the chapter on membrane electricity. Review those rules before proceeding if you are not comfortable with them. (You can also find most of them summarized in a separate section at the end of this chapter “Terms used in ion electricity.”)
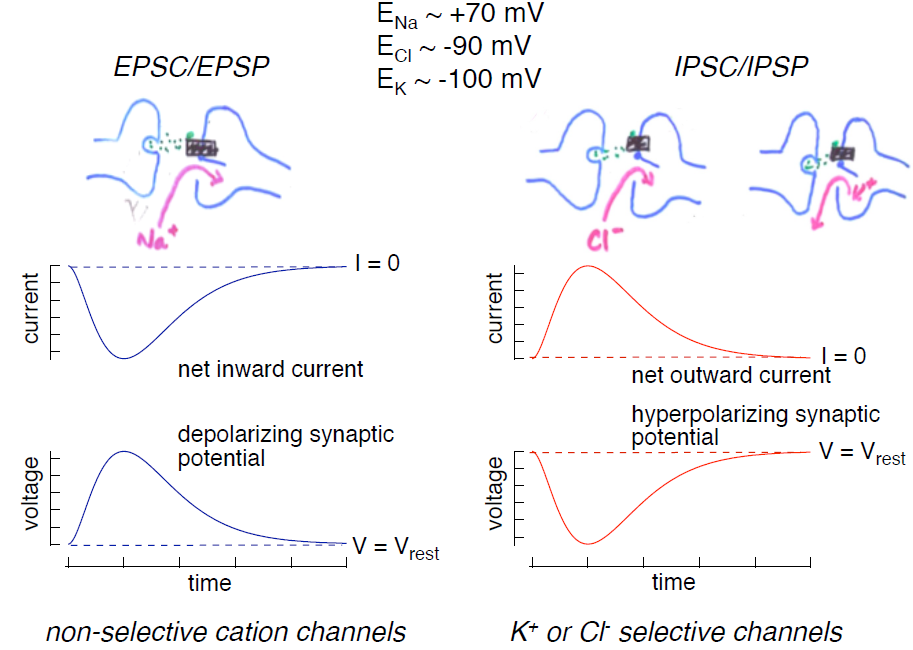
1. Excitatory postsynaptic potentials (EPSPs; Figure 3, left): Depolarizing excitatory postsynaptic potentials are generated by the opening of non-selective cation channels; current through these channels reverses at a potential of 0 mV, significantly more depolarized than the cell’s resting potential. Hence opening of these channels produces an inward current (referred to as an excitatory postsynaptic current or EPSC). This inward current produces the depolarizing excitatory synaptic potential, the EPSP.
2. Inhibitory postsynaptic potentials (IPSPs; Figure 3, right): Hyperpolarizing inhibitory postsynaptic potentials are generated by transmitter-gated ion channels permeable to either K+ or Cl–. Since the normal resting potential is more positive than the equilibrium potentials for K+ and Cl–, opening either of these types of channels will produce an outward current (an IPSC) generated by either outward K+ or inward Cl– flow, and this outward current will hyperpolarize the cell.
Excitatory and inhibitory synapses in action
The knee jerk reflex provides a nice example of the importance of EPSPs and IPSPs. You will learn about these spinal reflex pathways in more detail later; here we focus on the role of EPSPs and IPSPs. Extension of the lower leg involves increasing activity in the quadriceps muscles and decreasing activity in the hamstring muscles. The knee jerk reflex can be triggered when a reflex hammer imparts a small stretch to the quadriceps muscles; the stretch activates attached stretch receptors, producing action potentials in the sensory afferent nerve fibers innervating the stretch receptor. These afferent nerve fibers make excitatory synapses in the spinal cord both onto extensor motoneurons innervating the quadriceps and onto inhibitory interneurons in the spinal cord. Each of these afferent synapses produces EPSPs resulting in propagated action potentials. In the extensor motoneurons, APs lead to contraction of the quadriceps.
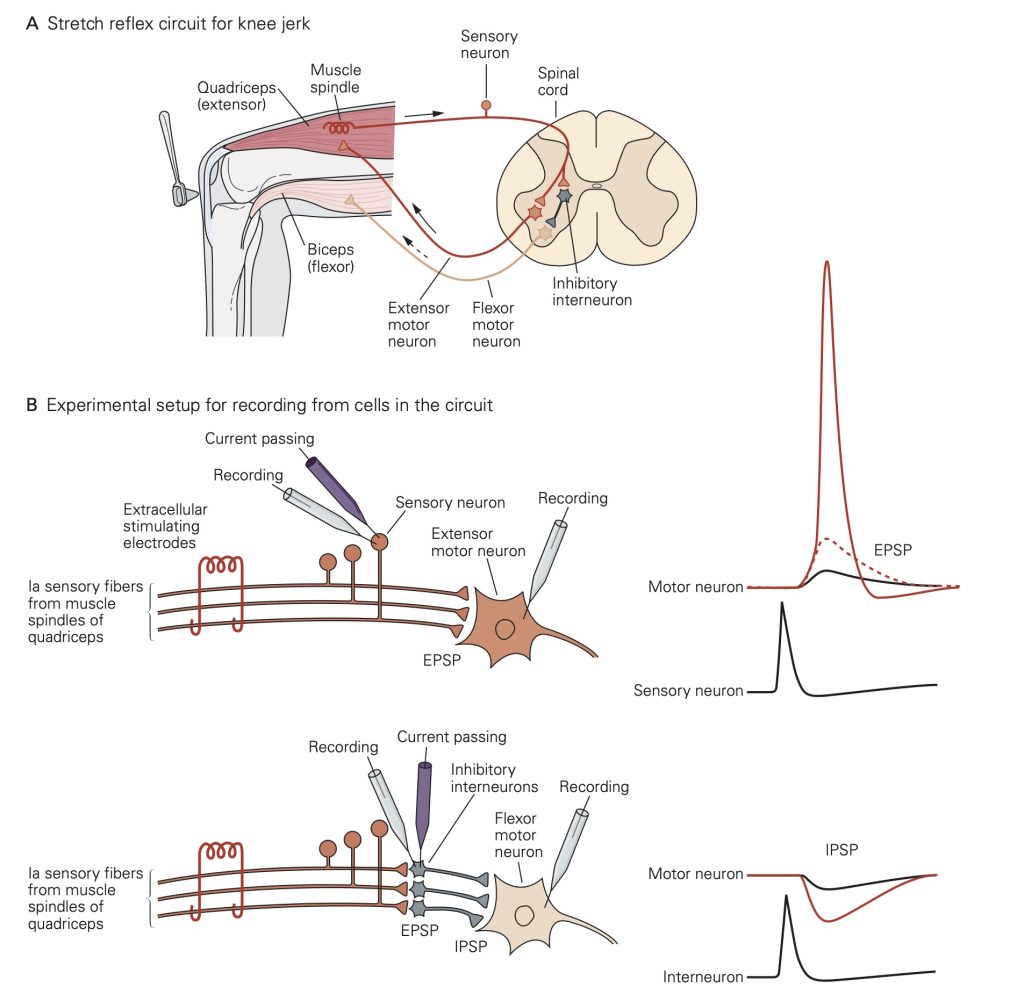
EPSPs in the inhibitory interneurons increase the rate at which they generate action potentials. The inhibitory interneurons make inhibitory synapses with the flexor motoneurons innervating the hamstrings. Thus, action potentials in the inhibitory interneurons lead to IPSPs that will decrease activity in the flexor motoneurons (the motoneurons exhibit some spontaneous activity). The decreased flexor motoneuron activity decreases activity of the hamstrings.
Together, the increase in activity of the quadriceps and decrease in activity of the hamstrings leads to extension of the knee. The opposing changes in activity in the two muscle types in response to activation of the same stretch receptors illustrates the importance of having both excitatory and inhibitory synapses.
Learning Objective #4: Describe the mechanism by which neurotransmitter is cleared at chemical synapses.
Synaptic potentials vary widely in time course. The speed of synaptic transmission is influenced by: (1) the time required for transmitter to be released and diffuse across the synaptic cleft— which is typically extremely short, and (2) the time required to generate the postsynaptic conductance change—which is quite variable. As described above, rapid release of transmitter relies on the close proximity of the vesicle release sites and the Ca2+ channels that permit Ca2+ to enter the nerve terminal and trigger exocytosis. The time for diffusion across the synaptic cleft is minimized by the close proximity of pre and post-synaptic membranes.
Postsynaptic events substantially shape the time course of the synaptic potentials and in doing so introduce diversity in the time course of synaptic potentials. An important division is into fast versus slow chemical synaptic transmission:
Fast synaptic transmission
Neurotransmitter binds to and activates receptors that are ligand-gated ion channels – these are referred to as ionotropic receptors (Learning Objective #3). The lack of intermediate steps between transmitter binding and the conductance change leads to a brief synaptic potential (1–20 ms in duration typically).
Recovery of the synaptic potential is mediated by receptor desensitization and by clearance of released transmitter. Receptor desensitization occurs when the channel associated with the receptor closes even though transmitter is still bound to the receptor – a situation a bit like inactivation of voltage-gated Na+ channels. Transmitter clearance at neuromuscular junctions is achieved via degradation of ACh by acetylcholinesterase; this is atypical, however, and other types of released neurotransmitter are cleared by transporters. Transporters located on glial cells near the synapse play a central role in “reuptake” or clearance of released transmitter (Learning Objective #4). These transport proteins do not use ATP but instead obtain the energy they need to move neurotransmitter out of the synaptic cleft by allowing ions such as Na+ to flow down their electrochemical gradient; as a result, they are Na+-coupled cotransporters (see also the chapter on membrane transport). Several therapeutic drugs target these transporters (see below).
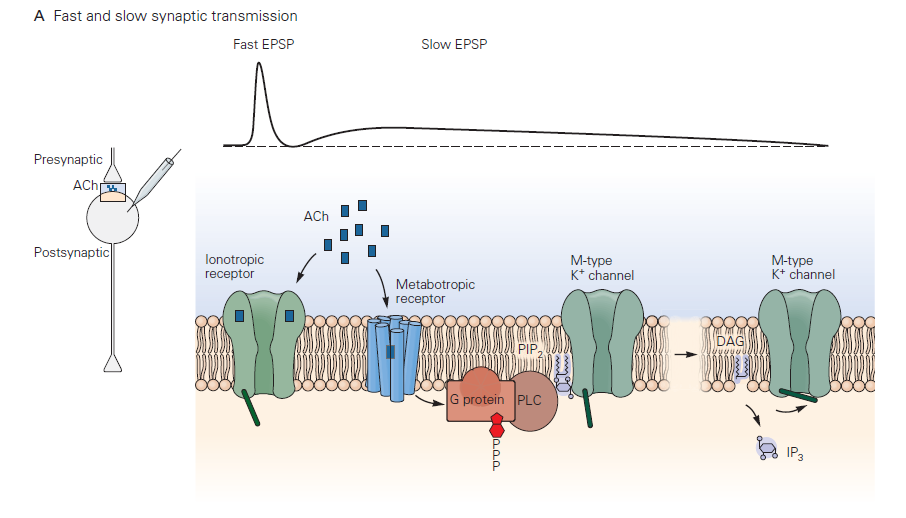
Slow synaptic transmission
This type of postsynaptic response mechanism differs radically from that in fast chemical transmission. Neurotransmitter binds to and activates a G-protein- coupled receptor (GPCR) – referred to by neurobiologists as a metabotropic receptor (Learning Objective #3). This leads to G-protein signaling and a cascade of biochemical events that may include opening or closing of ion channels, producing a slow synaptic potentials. The electrical response, as you would guess, takes longer to initiate and terminate than fast synaptic potentials due to the delays introduced by the chemical reactions comprising the G-protein cascade. Typical durations can be several to many seconds. A reason for the longer lasting synaptic potentials is that the biochemical events leading to conductance change can persist well after the transmitter has been removed from the synaptic cleft. The duration of slow synaptic potentials is determined by the time to shut off the activated G-protein signaling components. The G-protein needs to hydrolyze GTP to GDP, and downstream biochemical processes and second messengers need to return to the resting state. For more information about signaling through GPCRs, see also the chapter on G-protein Coupled Receptors.
Aside: Drugs acting on synaptic transmission. Many therapeutic and recreational drugs act on synaptic transmission. These have also been key tools in furthering our understanding of how synaptic transmission works. Essentially every component of the synapse is a target:
- Receptor enhancement: Benzodiazepines enhance effects of GABA. These drugs strengthen GABA synapses by causing the postsynaptic receptors to be more sensitive to GABA released by the presynaptic cell. These drugs are tranquilizers and may induce sleep.
- Receptor agonists: LSD mimics serotonin at serotonin receptors. The result may be hallucinations. Receptor agonists severely alter synaptic transmission by directly binding to and activating neurotransmitter receptors and bypassing transmitter release from the presynaptic cell.
- Receptor antagonists: Antipsychotics block dopamine receptors (e.g. haloperidol). Curare blocks nicotinic ACh receptor leading to muscle paralysis. These drugs suppress synaptic transmission by partially or completely blocking neurotransmitter receptors.
- Reuptake blockers: Most antidepressants inhibit serotonin uptake (e.g. Prozac); cocaine blocks norepinephrine, serotonin and dopamine uptake. These drugs permit released transmitter to remain in the synaptic cleft for a longer period of time and thus to elicit a stronger postsynaptic response. Unlike for receptor agonists, here the generation of a signal in the postsynaptic cell still relies on release of a chemical neurotransmitter.
- Block enzymatic degradation: Nerve gases, some insecticides, and research and clinical anticholinesterases inhibit degradation of ACh by acetylcholinesterase at the cholinergic junction. Like reuptake blockers, these drugs permit transmitter to remain in the synaptic cleft for a longer period of time. Low doses of such drugs may have therapeutic effects by enhancing the response to released transmitter (much like drugs that block reuptake mechanisms at other synapses). Nerve gases typically work by asphyxia as the muscles controlling breathing cease to be controlled.
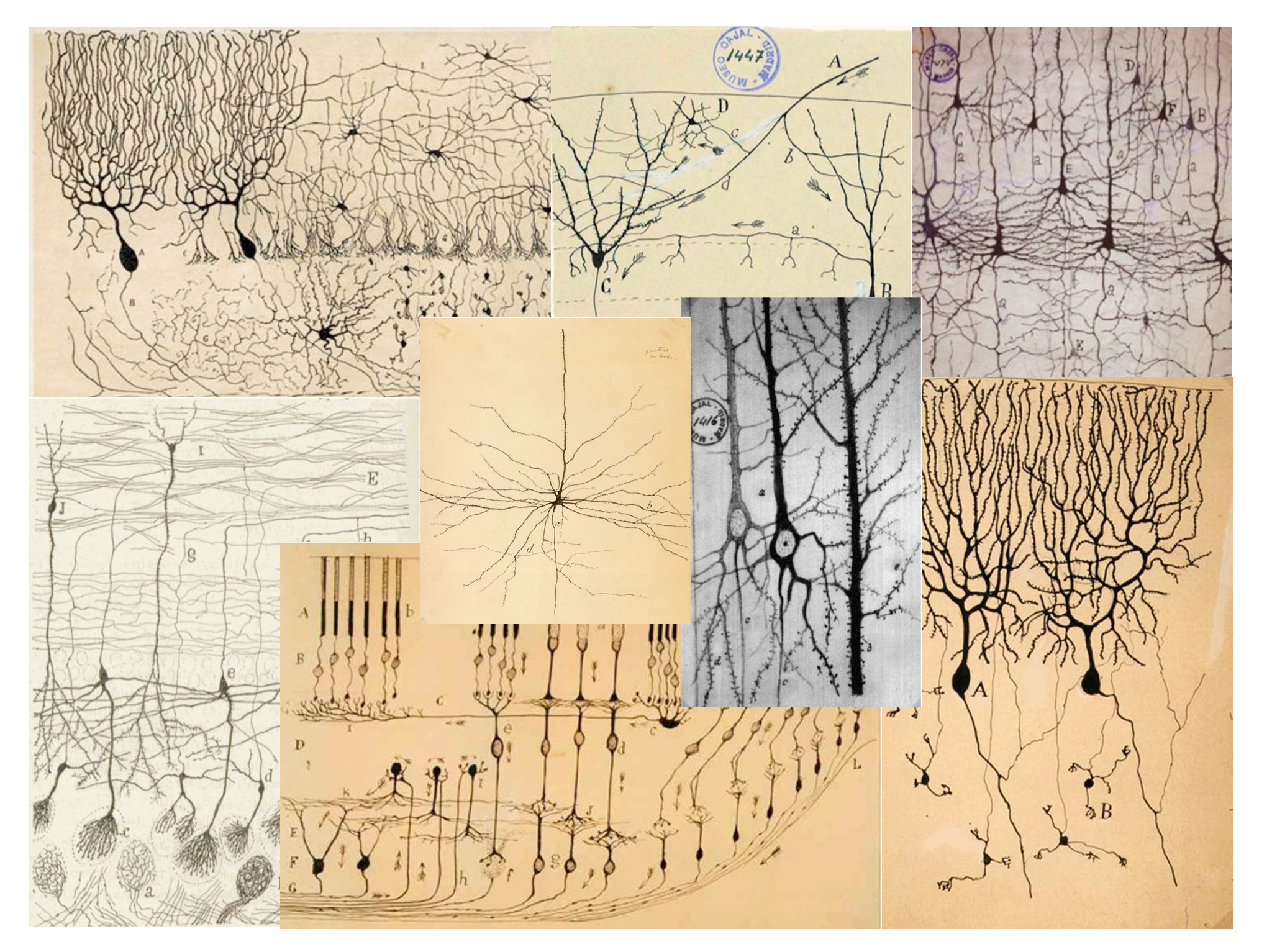
An important difference between synapses in the central nervous system and those at the neuromuscular junction is the number of excitatory inputs required to generate an action potential in the postsynaptic cell. At a healthy neuromuscular junction, a single presynaptic action potential reliably leads to an action potential in the muscle. A typical central nervous system neuron receives input from several thousand different neurons (convergence) and sends its outputs to several thousand neurons (divergence). These inputs are spread across the dendrites of the cells. You can appreciate the remarkable range of dendritic structures in Figure 6; these different structures are specialized for the precise function of the cell. Because the amplitude of a single synaptic input is typically less than 1 mV, multiple excitatory inputs must be summed or integrated to depolarize a neuron in the central nervous system to the firing threshold for generating an action potential. The term synaptic integration refers to the process by which multiple inputs are combined to control activity in the postsynaptic cell. This integration takes two forms:
• Temporal integration: integration of synaptic potentials from repetitive activation of one synaptic input. If the time between action potentials in the presynaptic cell is shorter than the duration of the synaptic potential produced in response to a single presynaptic action potential, the synaptic potentials produced by each action potential will begin to pile up, resulting in a larger response than that produced by a single presynaptic action potential. This process is referred to as temporal integration inputs originating at different times are combined or integrated.
• Spatial integration: integration of synaptic potentials from different synaptic inputs. Synaptic potentials produced at similar times from different synapses will be combined in the dendrites or at the soma of the postsynaptic cell and thus produce a larger response than that produced by activation of a single synapse. This is referred to as spatial integration because the inputs occur at similar times but at different spatial locations.
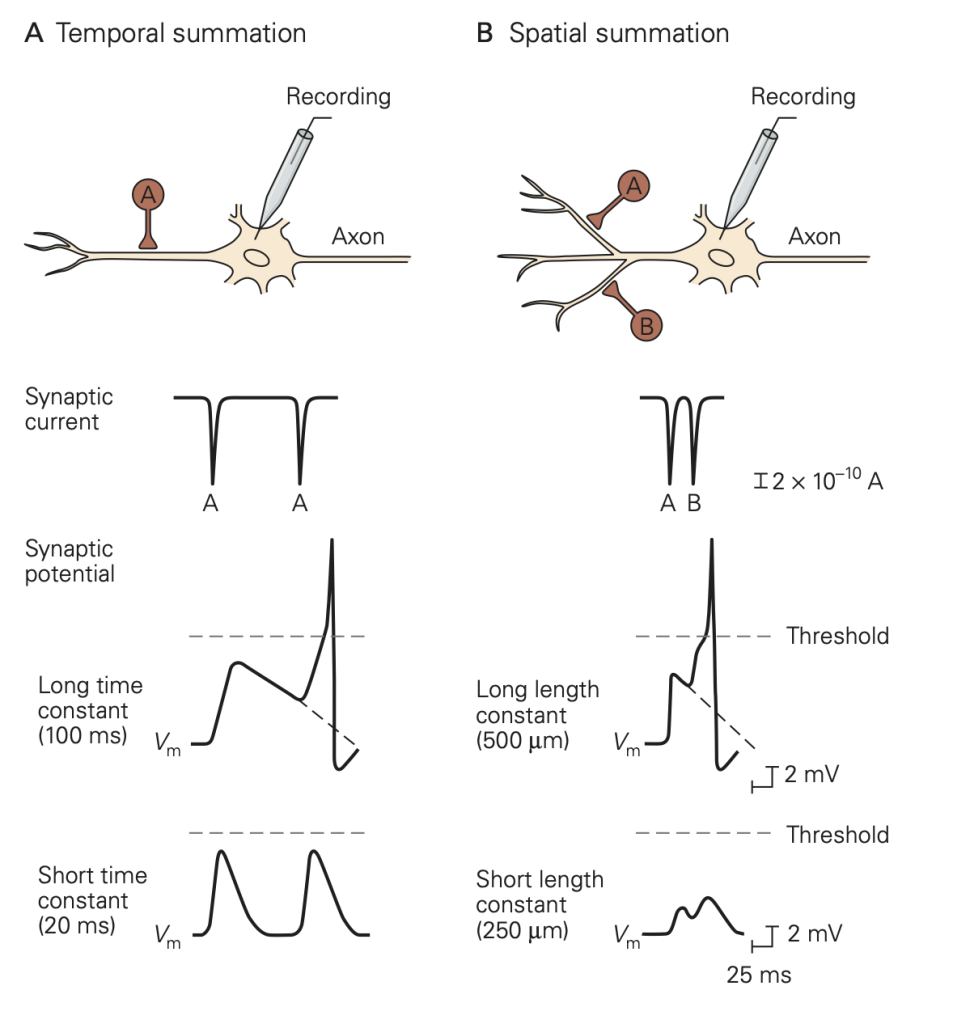
Pre- and post-synaptic inhibition
The massive convergence of inputs to a typical central nervous system neuron raises a conundrum: how does a cell receiving thousands of inputs (even if those inputs produce synaptic potentials less than 1 mV) maintain a reasonable firing rate? Fortunately, not all the inputs are excitatory, and indeed balancing excitatory and inhibitory inputs is critical to normal neural function. You can consider the neuron as a polling station where many voters are casting Yea and Nay votes to control action potential firing. The inhibitory signals that prevent runaway excitation are provided by inhibitory interneurons. These cells influence synaptic integration in two main ways.
• Presynaptic inhibition (Figure 8): In this case, activity in an inhibitory neuron (green in Figure 8) leads to activation of inhibitory synapses (which release GABA in the figure) made onto the presynaptic terminal (yellow). This activity reduces the amount of transmitter released by an action potential that subsequently propagates down the axon of the yellow cell and invades its synaptic terminal. A common mode of action is that the released inhibitory transmitter activates GPCRs, which initiate a G-protein cascade that inhibits presynaptic Ca2+ channels, causing fewer Ca2+ channels to open when the terminal is depolarized. This mode of inhibition requires that the (green) inhibitory neuron be active (i.e. generates an action potential) first, so that the green inhibitory synapse can be activated and exert its effect before the action potential arrives in the yellow synaptic terminal.
• Postsynaptic inhibition: Postsynaptic inhibition is a form of spatial integration. IPSPs generated by inhibitory inputs to a cell will fully or partially cancel EPSPs generated by excitatory inputs when they are combined in the dendrites or at the soma. Balancing of excitatory and inhibitory inputs in this context means that the average of all the EPSPs received by the cell tends to be cancelled by the average of all the IPSPs. Appropriate stimuli can disrupt this cancellation and cause either the EPSPs to win out, and the firing rate to increase, or the IPSPs to win out, and the firing rate to decrease. Thus, firing patterns of many neurons in the brain reflect changes in the balance of an ongoing barrage of excitatory and inhibitory synaptic inputs. As in a voting booth, the YES (excitatory) votes have to exceed the NO (inhibitory) votes for activity to increase. These balanced choices underlie the decisions made by your brain.
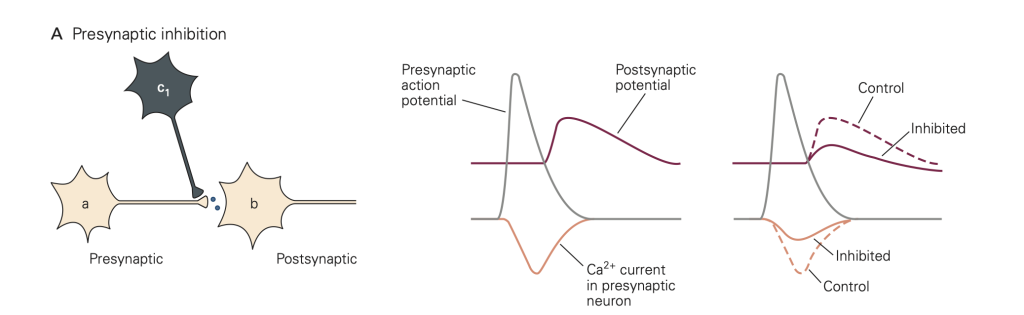
The second major form of synaptic transmission is mediated by electrical synapses. At an electrical synapse, signals are transmitted from one cell to another by direct current flow through structures called gap junctions (Figure 9). Electrical synapses are less common than chemical synapses in our brain, but are often found in sensory systems (like the retina) and in non-neural tissues like epithelia, the liver, and heart. They play an especially important role in synchronizing groups of cells. In nonneuronal systems, only the phrase gap junction rather than the word synapse is used for such common cell-to-cell communication. In evolutionary terms, gap junction structures are ancient forms of cellular communication.
Gap junctions provide a non-selective conductance path between the two cells, permitting exchange of ions, second messengers, and small molecules between the cells. They are often identified based on their ability to pass small tracer molecules such as the yellow dye illustrated in Figure 10; here the dye was injected into one of the cells, and passed, via gap junctions, into the others. Because gap junctions provide a non-selective path for current to flow, they tend to equalize the voltage in the two cells. This is illustrated in Figure 11. If V1 > V2, current will flow from cell 1 to cell 2 and bring the voltages closer together. Vice-versa if V2 > V1. This property makes electrical synapses particularly good at synchronizing the electrical activity in populations of cells, a property essential for cell-to-cell propagation of action potentials in cardiac muscle.
Indeed, many cardiac diseases are marked by a disruption of normal patterns of gap junctions between the cells. Unfortunately, we have few good (i.e. specific) drugs that act on gap junctions. This hampers study of their function and the treatment of disorders associated with them.
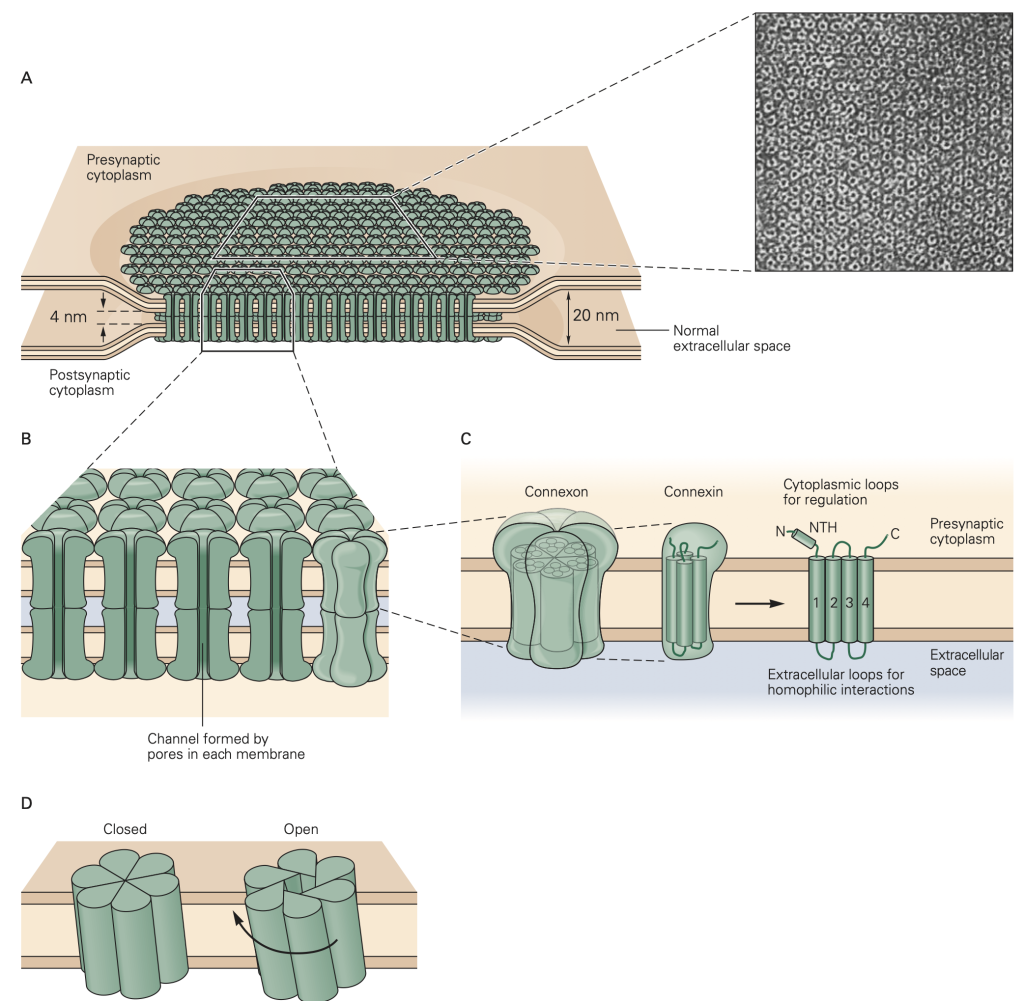
The electrical synapse relies on current from one cell causing a voltage change in the connected cell. For this form of synaptic transmission to be effective, the two cells must have similar resistances. If the cells have very different resistances, current passed from the high resistance cell to the low resistance cell will not be very effective in changing its voltage. As an analogy, think of pressing a hot needle up against a cannon ball; the needle doesn’t do much to heat the cannon ball because of its small size. A hot cannon ball, pressed up against the second cannon ball, would be much more effective. These reasons, for example, make gap junctions unsuitable at our neuromuscular junctions where motoneuron axons are small compared to the muscle cells that they innervate.
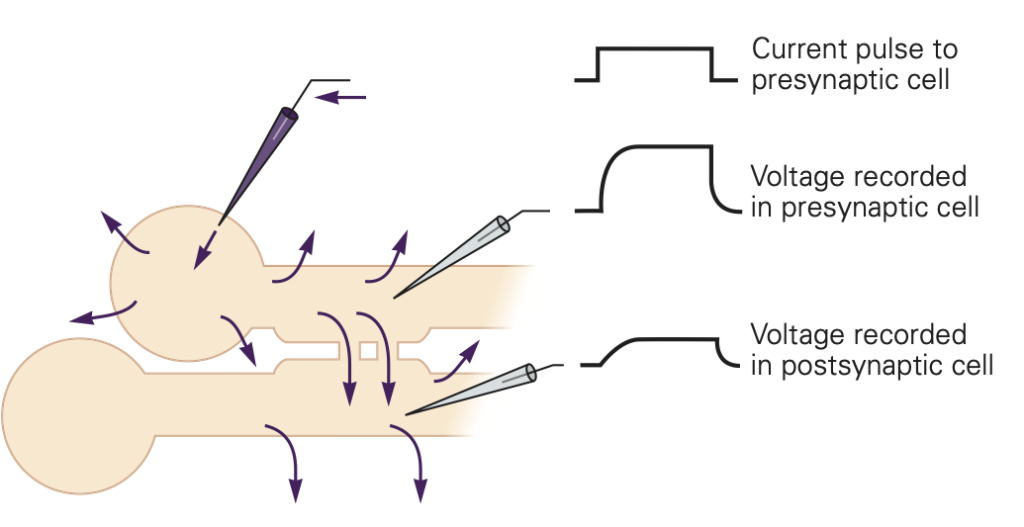
Conclusion
To send and coordinate messages, the nervous system uses propagated action potentials in axons, chemical or electrical communication to the next cells at synapses, and both inhibitory and excitatory postsynaptic potentials to allow complex integration and decision making–computations that revolve around reaching firing threshold.
Terms used in ionic electricity
- Charge: Ions have a charge given in multiples of one elementary electron charge. Cations (Na+, K+, Ca2+) are positively charged and anions (Cl–) are negatively charged.
- Two forces that move ions:
- Charges are moved by the force of electric fields: Opposites attract, so cations (positively charged) move towards a negative pole, and anions towards a positive pole.
- In addition, ions are moved by diffusion (thermal agitation) down their concentration gradients–even in the absence of electric fields.
- Current: A net movement of charge is an electric current measured in amperes (A) and symbolized by I. Thus, a net flow of ions is an ion current The direction of current is defined as the direction of positive charge movement, so in neurophysiology, a positive current means positive charges moving out of a cell, an outward current.
- Voltage (synonymous with potential): If cations are removed from a compartment (the cell), the compartment becomes more negative, a negative voltage will be set up inside the cell. A voltage is measured in units of volts (V) and symbolized by V. Positive charges want to move to regions of more negative voltages in the absence of other forces.
- Conductance: Membrane conductance describes how easily ions can cross the membrane. Conductance, symbolized G, is proportional to the number of ion channels open. Each open channel contributes a small conductance to the total. (A closely related inverse concept, resistance, expresses how hard it is for ions to move. Mathematically, resistance is the reciprocal of conductance (1/G). An insulator has high resistance and low conductance.)
- Capacitance: Electrical capacitors store charge. Two conductors separated by a thin insulator (membrane) form an electrical capacitor. The magnitude of a capacitance (symbolized C) is defined as the number of charges that you have to move to achieve a certain potential change. Therefore, the capacitance of cell membranes tells us how many ions have to be moved across the membrane to change the membrane potential during signaling.
- Equilibrium potential for an ion: The membrane potential at which the electrical force and the diffusion force (due to the concentration gradient) exactly cancel for that particular ion. At the equilibrium potential there will be no net force on that ion across the membrane.
- Reversal potential for an ion channel: The membrane potential at which no net charge movement occurs in that ion channel. The channel may not be perfectly ion selective so that at the reversal potential one kind of ion may move one way and another may move the opposite way, but the net effect is no charge movement. When only one kind of channel is open, the ion flow in that channel tends to move the membrane potential to the reversal potential for that channel. Equilibrium potential is a thermodynamic property of an ion, given its gradient. Reversal potential is a property of an ion channel, given its ion selectivity and the gradients of the permeant ions.
Feedback: