Cell Membranes and Transport
Fred Rieke and Bertil Hille
Session Learning Objectives and Quick Synopses
1. Describe the structure and topology of cellular membranes.
Cell membranes surround the cell and surround each organelle in the cell. They are formed by bilayers of phospholipids. The phospholipid tails form a hydrophobic (water-fearing) core between two hydrophilic (water-loving) layers formed by the phospholipid head-groups. Molecules that are lipid soluble dissolve in and pass readily through the bilayer. In addition, integral and peripheral membrane proteins are embedded in the lipid bilayer. They confer additional membrane properties through their functions as receptors, enzymes, or permeation pathways.
2. Contrast ion channels and ion transporters and the forces that drive them.
Ion channel proteins are gated pores in the membrane that allow selected ions to flow down their electrochemical gradients. In ion channels, the ions are driven only by their own concentration gradients and by the electric field acting on them. We call this flow passive. Other transport proteins act more like enzymes that couple the movement of one kind of ion or solute to energy derived either from the flow of another kind of ion or directly from the hydrolysis of ATP. Often these transporters can establish gradients of ion concentration. We call this active transport. It is primary active transport when the pump is driven by ATP, and secondary active transport when the energy comes from another ion gradient.
3. Know the normal balance of Na+, K+, Cl– and Ca2+ with respect to the plasma membrane.
As a result of active transport, Na+, Ca2+, and Cl– are more abundant outside of the cell in serum, whereas K+ is more abundant inside the cell in cytoplasm (Table 2). These non-equilibrium distributions are essential for many cellular responses including electrical excitability.
Cell membranes
All cells are surrounded by a plasma membrane that forms the boundary between the living cytoplasm and the extracellular space. The membrane retains the cytoplasmic contents and prevents intrusion by most molecules outside the cell. The membrane is flexible and semi-fluid, conforming dynamically to the changing forces of intracellular cytoskeletal proteins and the extracellular matrix and substrate. Red blood cells, for example, take their biconcave disk shape from their specialized spring-like intracellular cytoskeletal network. Nevertheless, they readily bend and squeeze unharmed in transient contortions as they rush through narrow capillaries. Inside nucleated cells, other membranes define organelles like the mitochondria, the nucleus, and the endoplasmic reticulum. The plasma membrane and the intracellular membranes share a common basic architecture and a common origin. They are made of a molecular bilayer of lipids peppered with membrane proteins. We start by discussing the lipid component and then return to the proteins.
Membranes form a hydrophobic lipid bilayer
Typical membrane lipids are phospholipids and cholesterol. These molecules are primarily hydrophobic (“water fearing”) but bear a charged or polar group at one end that is hydrophilic (“water loving”) (Figure 1). We call this dual nature, amphipathic (“both preferences”). Soaps and detergents are also amphipathic. This allows them to bring greasy substances into solution in water (the cleaning action of soaps, for example, relies on breaking grease up into small droplets in water).
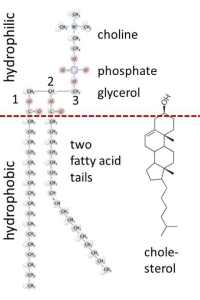
In the structure of phospholipids, we see two hydrophobic fatty acid chains attached to a glycerol scaffold at the glycerol 1- and 2-positions (Figure 1). The glycerol 3-position carries the hydrophilic phosphate and head group. Common head groups include (Figure 2) serine, ethanolamine, choline, and inositol, each with its own special roles and forming phospholipids with names like phosphatidyl serine, phosphatidylinositol, and so forth. Amphipathic phospholipids spontaneously form a lipid bilayer made of two lipid leaflets as a thin flexible sheet (Figure 3). This is a minimum energy state for phospholipids in water because it maximizes the interactions of the hydrophobic fatty acid side chains with each other and away from water, and it maximizes the interaction of the hydrophilic head groups with water.
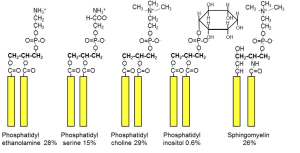
At physiological temperatures, the lipid side chains are free to undergo rapid spontaneous thermal motion, waving around in a fluid-like state while still remaining anchored to the glycerol, which carries the head group. In addition, each phospholipid molecule experiences spontaneous thermal rotation, stochastically spinning around its long axis, as well as lateral diffusion by Brownian motion in the plane of the membrane. All these motions make the lipid bilayer an ultrathin flexible, oily fluid. The thickness is just twice that of the two opposing fatty acid leaflets, about 3 nm, far thinner than the wavelength of light and therefore resolvable only by electron microscopy.
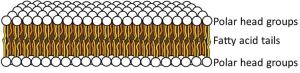
Passive permeability of the bilayer
Some molecules easily cross the lipid bilayer membrane and some do not (Figure 4). Permeability is a measure of how easily molecules cross the membrane. Key permeability properties of cell membranes are well understood if we remember that the lipid bilayer is like a thin sheet of oil. Hydrophobic molecules presented in the aqueous phase, being lipid soluble, pass easily into the oily lipid bilayer and cross the membrane barrier. Illustrative examples would include aspirin, steroid hormones, O2, CO2, and NO (Box A). We say the membrane is permeable to these hydrophobic molecules, which is good in these cases since their physiological actions require them to enter cells from the outside. Thus, the steroid hormone estrogen is made in the ovaries but has to act on receptors in the nucleus of cells throughout the body. In all respiring cells, O2 has to enter the cell continuously, and CO2 has to leave. Similarly, O2 has to enter red blood cells in the lungs and be delivered to tissues in the capillaries during every circuit of the circulation, and the reverse for CO2. Each of these steps requires the hydrophobic steroid or gas molecules to dissolve in the plasma membrane and to pass across it and emerge on the other side, a process that takes only a small fraction of a second. For the same reasons, some degree of hydrophobicity is essential in the design of new drugs that are intended to be taken orally so that they can be absorbed across cells of the intestinal wall and cross into target tissues.
On the other hand, molecules that are polar (sugars) or charged (ions) are hydrophilic and do not enter into oil. They are reluctant to leave water and do not enter or cross the lipid bilayer easily. Examples include Na+, ATP, and glucose (Box A). Cell membranes are relatively impermeable to them, which allows the cell to develop ion gradients across membranes and to retain its high-energy molecules, metabolites, and genetic material inside. Many important physiological responses involve changes of membrane permeability to charged and polar molecules. In electrophysiology we will see that the ion-impermeable lipid bilayer can be thought of as an electrical insulator. It allows a small charge imbalance across the membrane to generate an electrical potential difference across the membrane – the membrane potential. The electrical responses of cells involve changes of permeability to ions and ion movements through the carefully orchestrated opening and closing of ion channels in the plasma membrane. In this way, as we shall see later, the plasma membrane is the seat of all electrical signaling in nerve and muscle.
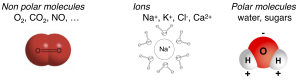
Proteins add functions to cell membranes
A more complete view of biological membranes would show a thin lipid sea with many proteins inserted and floating in it (Figure 5). Some proteins, the integral membrane proteins, have one or more hydrophobic transmembrane segments and never leave the membrane (Box B). Others, the peripheral proteins, bind to other membrane proteins and lipid head groups but do not have any transmembrane segments. They may associate and dissociate dynamically from the membrane compartment depending on the state of the cell.
Almost all integral membrane proteins are initially inserted into membranes of the endoplasmic reticulum (ER) during protein synthesis as the nascent peptide chain is lengthened by protein translation. The ER membrane then buds off a membrane vesicle that passes to the Golgi with its new membrane proteins. Eventually, more budding from the Golgi sends a vesicle further to, e.g., the plasma membrane.
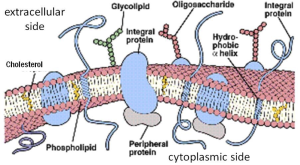
Details of membrane origin and traffic will be discussed in a separate session emphasizing cell biology of membrane budding and endo- and exocytosis. They explain how all membranes have a similar architecture since they derive from the same source. Membrane proteins contribute many biological functions to the membrane including transport and enzymatic catalysis (Box B). Although the basic architecture of the membrane is conserved, the different membranes of a cell are specialized by having different sets of functional proteins to allow them to perform different functions, so both at the level of gene expression and in the events of membrane traffic, decisions are made as to which protein belongs where.
Learning Objective #2. Contrast ion channels and ion transporters and the forces that drive them.
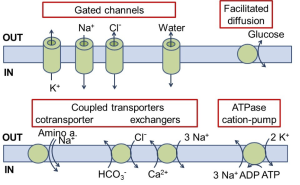
Now we will emphasize certain transmembrane proteins that facilitate movement of ions across the membrane, sometimes coupling with transport of other small molecules. One can consider them catalysts that enable regulated transmembrane movements of a small select number of molecules. They include ion channels, coupled transporters, and ion ATPase pumps (Figure 6). We introduce them superficially first.
(A) The ion channels are the simplest. When they are open, they act like a pore that allows ions to flow. They have ion selectivity – a preference for certain ions. The direction of ion flow is set entirely by two thermodynamic forces on the ions: (i) the ion concentration gradient across the membrane, and (ii) an electrical force if there is a difference in electrical potential across the membrane. We can say that the direction of ion flux is thermodynamically downhill, and, more technically, we say it is down the electrochemical gradient. The ion channels in Figure 6 are selective for K+, Na+, or Cl– and would be called K+ channels, Na+ channels, or Cl– channels. Some ion channels are less selective, passing a range of small cations or a range of small anions. Ion channels have an additional feature: most of them open and close their pore in response to stimuli such as a neurotransmitter, a voltage change, heat, or an intracellular second messenger. We say they are gated. This gating allows them to react dynamically to other stimuli. The figure also shows a water channel, a pore that allows osmotic flow of water across the membrane, e.g. in the kidney. As a peek forward to the next few weeks, Table 1 lists the properties of ion channels you will be encountering soon.
(B) The next category in Figure 6, the coupled transporters, couples the movement of one molecule (here an organic solute or bicarbonate or Ca2+) to the downhill flow of another (Na+ or Cl–). The action is like that of an enzyme that in this case has two substrates. Nothing happens until both substrates are present. They bind and induce conformational changes of the protein that exposes each of them to the opposite side of the membrane. Such transporters are sometimes called “carriers.” The first example shown is a Na+-coupled solute transporter, of which there are many types (for sugars, amino acids, neurotransmitters, etc.). Here Na+ and the coupled solute move in the same direction into the cell, so it is called a co-transporter. These cotransporters use the free energy of Na+ allowed to flow downhill (down its electrochemical gradient) into the cell, to drive the cotransport of the other substrate. They can drive uphill transport of solutes, creating a concentration gradient. Such a process is called secondary active transport, an active transport that does not use ATP as its direct source of energy. It is analogous to using the cascading of water over a waterfall (Na+ entry) to do some work (solute transport).
The second group of coupled transporters exchanges ions from opposite sides of the membrane. Shown are examples of Cl–/bicarbonate exchangers and Na+/Ca2+ exchangers. All these coupled transporters are fully reversible, operating in whichever direction thermodynamics dictates, and are capable of secondary active transport. For completeness, we also mention a type of transporter that has only one substrate and no coupled ion flow (Figure 6). This transport process, called facilitated diffusion, is by necessity thermodynamically downhill for the single substrate. A notable example is the Glut4 glucose transporter inserted into the plasma membrane by the action of insulin to allow passage of glucose in either direction.
(C) The final example in Figure 6 is an ion pump that couples transport of Na+ and K+ to the free energy of hydrolysis of ATP, the metabolic dollar bill. Now both ions are moved uphill (in opposite directions) at the direct expense of metabolic energy. Such pumps that consume ATP directly are said to perform primary active transport. They generate the ion gradients across the membrane that are important for ion movements in coupled transporters and in ion channels. Before considering how the pumps work, we will discuss the ion imbalances that these pumps set up.
Four ion gradients
Gradients of ions across the plasma membrane are key for physiology and medicine–indeed for life. The word gradient means a difference in concentration on the two sides of the membrane. Recall that salts like NaCl dissociate in water into the free cations and anions – Na+ (“sodium ion”) and Cl– (“chloride ion”) in the case of NaCl. This makes an electrolyte solution. With respect to ions, our plasma (the extracellular medium for cells) is like a dilute version of seawater, reflecting the origins of animals in early oceans. As in seawater, the concentrations of Na+ and Cl– are high and K+ is low (Table 2). In contrast, inside the cell, the Na+ is low, Cl– is often low, and K+ is high. Resting cellular free Ca2+ is extraordinarily low, making Ca2+ a good ion for intracellular signaling as we will see later. Note that the table has a footnote explaining two conventions for the units of extracellular concentration that will be important for you as a clinician. Here we will use international scientific units, but in the clinic you will use the other. Shortly we shall see that ion gradients allow cells to make electrical signals. Later curriculum blocks show how ion gradients help to regulate cellular and body volume and to transport essential metabolites into cells by Na+-coupled transporters. Right now, it would be important to commit to memory the direction of these four important cellular ion gradients, remembering which ions are high outside, and which are high inside. These gradients are an essential concept for understanding electrical excitability and cell physiology.
Ion pumps make ion gradients
The existence of ion gradients tells us that the cell is not at equilibrium and that some work has been done to make such an imbalance. As is always the case, doing work requires expenditure of energy; this is the job of the ATPase ion pumps. In cell membranes, there are several pumps in this protein/gene family specialized for active transport of Na+, K+, Ca2+, and protons. We start with the Na+/K+-ATPase, sometimes just known as the Na+-pump for short.
Pumps are integral membrane proteins; more specifically, they are enzymes. Like other enzymes, they have a reaction velocity that increases with substrate concentration and that saturates when the substrates are abundant. Also, their reaction is cyclic with substrates binding and products unbinding in a fixed and cyclic stoichiometry. The unusual feature compared with most enzymes is that the substrate is picked up in one compartment and through a conformational change the product is released in a different compartment. They operate across membranes. The reaction for the Na+/K+-ATPase in the plasma membrane, already diagrammed in Figure 6, can be written as a chemical formula:
E + 3 Na+i + 2 K+o + ATP -> E + 3 Na+o + 2 K+i + ADP + P
where E stands for the enzyme, the numbers indicate the stoichiometry of the reaction, subscript “o” means outside the cell, and subscript “i” means inside the cell, except that Pi is the conventional abbreviation for “inorganic phosphate.” Let’s turn this into words: 3 Na+ ions are moved from the inside to the outside, 2 K+ ions are moved from the outside to the inside, and 1 ATP is broken down to ADP plus inorganic phosphate in the cytoplasm. Some known substeps of the pump cycle can be represented by a cyclic cartoon (Figure 7). Because this pump operates all the time in all our cells, the cytoplasmic Na+ is removed and replaced by K+, creating and maintaining the Na+/K+ gradients of Table 2. Some cells, like kidney cells and most excitable cells, must move ions rapidly to accomplish their transport and excitability functions. They have many copies of the Na+-pump in their plasma membrane. Others, like red blood cells, have very few ion channels and can get by with a low density of Na+-pumps. Red blood cells therefore do not need to consume glucose and produce ATP as rapidly as a kidney cell would.
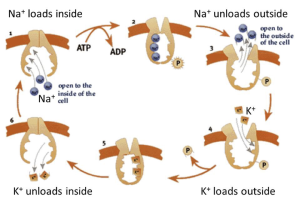
This pump can be blocked by cardiac glycosides like digitalis (related to Digoxin used clinically) and ouabain. When this is done experimentally, the ion gradients of the cell gradually run down over hours. Such experiments also show that as much as 25-40% of the energy metabolism of a kidney cell is devoted to providing the ATP for the Na+/K+-ATPase. Clinically, digitalis analogs are used at very low concentration to reduce the Na+ gradient just a little in the heart. As you will learn later in the Cardiovascular Block, a decreased Na+ gradient reduces the activity of the Na+/Ca2+ exchanger (see Figure 6), which is powered by the Na+ gradient. Therefore, some additional cytoplasmic Ca2+ accumulates inside the cardiac cells, boosting muscle contraction and helping to ameliorate congestive heart failure.
Practice questions and review topics:
Optional digoxin practice case
Patient Bob Jones is a 68-year-old man who suffered a heart attack 6 months ago, and as a consequence now has severe systolic heart failure (weakened pumping function of the heart muscle). Initial medications prescribed for this have resulted in only slight improvement of the heart failure symptoms, so Bob’s physician decides to add digoxin to the medication regimen.
Digoxin is a drug in the class called cardiac glycosides, which are derived from the foxglove plant and have great historic importance in medicine as they have been used for hundreds of years to treat heart failure. Newer drugs developed in recent decades have now become the mainstay of treatment for heart failure, but digoxin is still used in certain circumstances.
Among other effects, digoxin at optimal doses increases the force of contraction of heart muscle. The following questions will help you understand the physiology behind this therapeutic effect. Note: In a few weeks, you will be learning that intracellular Ca2+ triggers contraction of cardiac and skeletal muscle.
- Review the function of the Na+-K+ ATPase pump.
- How would inhibiting this Na+-K+ pump influence ion distribution?
- How would inhibition of the Na+/K+ ATPase influence forces contributing to ion movement across the plasma membrane?
- How might this decreased Na+ gradient influence function of a secondary active transport system?
- Review the mechanism of action of the Na+-Ca2+ exchanger.
- What would be the result on intracellular Ca2+ concentrations if activity of the Na+/K+ ATPase pump is slowed?
- Predict how this change could be useful in the treatment of cardiac failure.
- Now put together the physiologic processes discussed in this case, to explain the mechanism by which digoxin augments the force of myocardial contraction.
Feedback: