Action Potential Propagation
Fred Rieke
Learning Objectives and Quick Synopses
1. Describe how changes in resting membrane potential affect the action potential.
Changes in resting potential impact action potential generation in two ways: (1) manipulations that make the resting potential farther from threshold can mean that a larger stimulus is required to reach threshold; (2) manipulations that make the resting potential considerably more positive can cause failure of Na+ channels to recover from inactivation.
2. Explain the propagation of an action potential down an axon and describe how the refractory period contributes to this.
The propagating action potential depolarizes the axon in front of it, bring it to threshold so it too can generate an action potential. The propagating action potential leaves in its wake an area of membrane that is still refractory, and this prevents the action potential from propagating in both directions along the axon.
3. Describe the importance of myelination for action potential propagation.
Myelin decreases the membrane capacitance and increases its resistance. Both of these mean that less current is lost as the action potential propagates. This enables “saltatory conduction” wherein the action potential is regenerated at specific sites along the axon that lack myelin; transmission between these sites is passive (without regeneration).
Overview
In the Action Potential, Threshold, Refractory Period Chapter we studied how the gating of Na+ and K+ channels produces the action potential. Here we study two additional aspects of action potentials: (1) how changes in resting potential alter excitability – i.e. the number of action potentials that result from a given input to a cell, or alternatively how likely a small input is to generate an action potential; and, (2) how action potentials propagate down axons. Both of these are implicated in disease.
A key fact about the threshold for action potential generation is that it is not determined as a fixed voltage relative to the resting potential, but is instead determined by the voltage at which enough Na+ channels open to enter the positive feedback Hodgkin cycle. Thus threshold is determined by the number of available Na+ channels and their voltage dependence. Manipulations that change the resting potential can also change the voltage difference between it and action potential threshold; this in turn changes excitability by either bringing the voltage closer to threshold or moving it farther from threshold. Manipulations that cause a cell’s resting potential to be farther from the threshold (i.e. that make the resting potential more negative) decrease excitability – i.e. the cell is less likely to generate an action potential for a given stimulus. Manipulations that produce modest depolarization cause a cell’s resting potential to be closer to threshold for action potential generation and hence increase excitability — i.e. the cell is more likely to generate an action potential for a given stimulus.
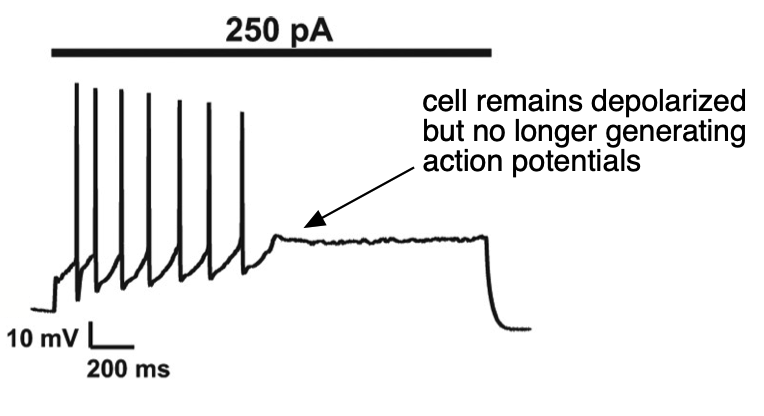
More extreme depolarization can completely suppress action potential generation as a cell goes into “depolarization block” (Figure 1) – in this case excitability goes to zero since the cell cannot generate an action potential. Depolarization block occurs when the voltage is sufficiently depolarized to suppress recovery of Na+ channels from inactivation (remember that the h or inactivation gate resets very slowly at potentials positive to the resting potential, see the Action Potential, Threshold, Refractory Period chapter, Figure 4). This means that there are not a sufficient number of Na+ channels available for opening for a cell to enter the Hodgkin cycle and generate an action potential. Such depolarization block persists until the voltage hyperpolarizes sufficiently for recovery of Na+ channel availability.
Ion channel mutations are another important cause of changes in excitability. Some of these mutations directly alter key components of the machinery described above for action potential generation. For example, pacemaking in the heart depends on the duration of the cardiac action potential, and mutations that alter inactivation of Na+ channels can disrupt pacemaking (e.g. in some forms of long QT syndrome). Other mutations can alter the resting potential. Cl– channels, along with the usual K+ channels, play an important role in setting resting potential in muscle. A clinically important example is loss-of-function mutations in chloride channels in myotonia congenita, which can depolarize the muscle resting potential and increase excitability. As a result, muscle contraction can be substantially prolonged, causing transient paralysis (google ‘myotonic goats’ for some examples). Mutations in “leak” K+ channels (remember from the Action Potential, Threshold, Refractory Period Chapter that these are distinct from the voltage-activated K+ channels activated during the action potential) similarly can alter resting potential and excitability.
Changes in the distribution of ions across the membrane can also change excitability. A clinically important example is hyperkalemia — an elevation in extracellular K+ concentration. Since K+ plays a key role in setting the resting potential, and since EK depends strongly on the external K+ concentration, relatively small increases in extracellular K+ can alter the K+ equilibrium potential and depolarize the membrane sufficiently to have critical effects on excitability — including causing alterations and even failure of action potential generation in the heart or other muscles. The sensitivity to resting potential to changes in extracellular K+ is one reason you will want to watch it closely when you get lab results back on your patients!
A simple exercise to check your understanding
The mechanisms we discussed in the Action Potential, Threshold, Refractory Period chapter account for the generation of an action potential, but not how action potentials are propagated in one direction down an axon. Action potentials are initiated at the axon hillock, a region of the axon near the soma with a high density of Na+ channels and hence a low threshold for generating action potentials (Figure 2); a higher density of Na+ channels means more channels are available to open, and as a result the positive-feedback Hodgkin cycle is entered at more hyperpolarized voltages. Action potentials then propagate down the axon as a wave of depolarization (Figure 3).
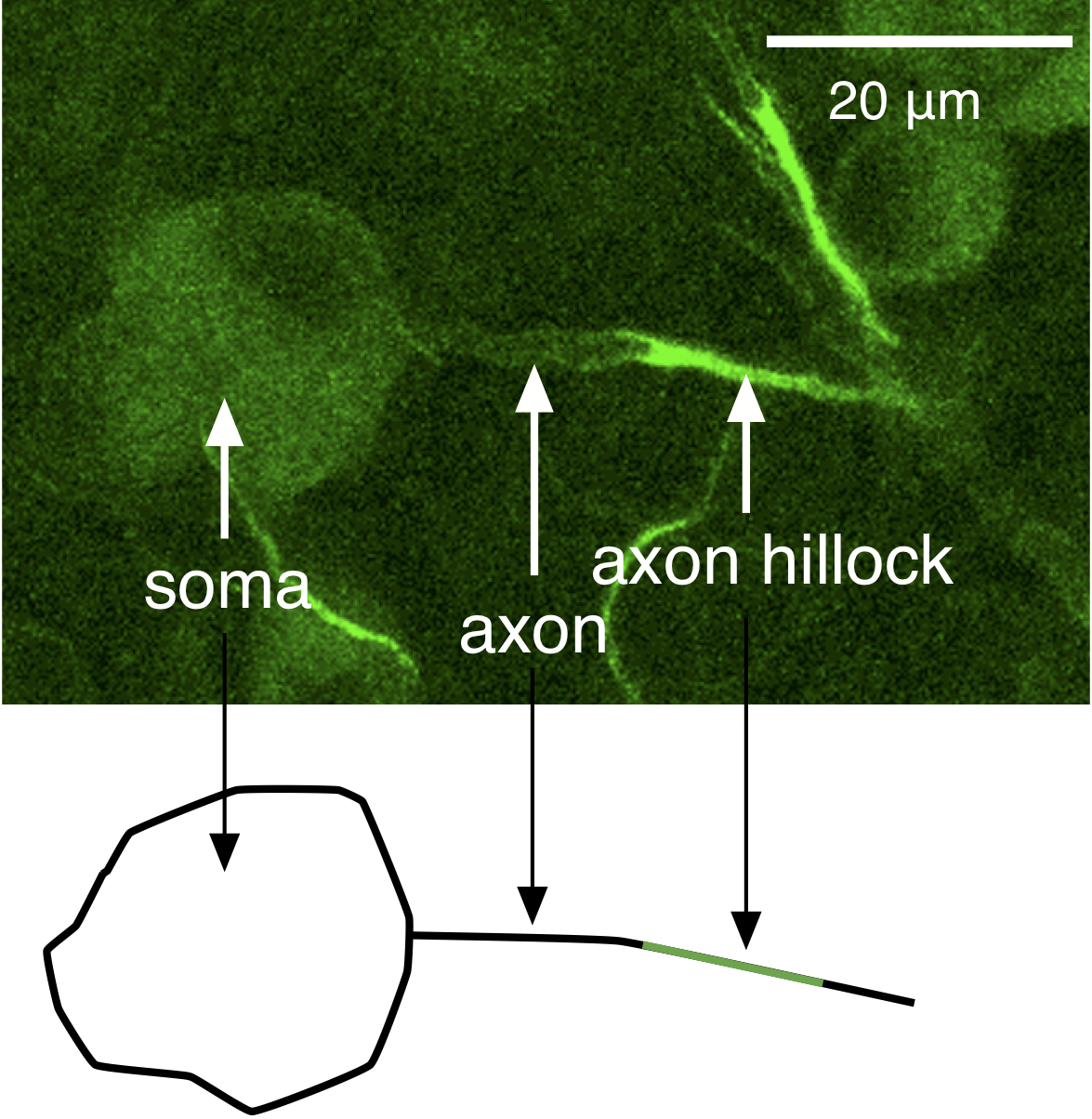
The propagating action potential sets up a spatial profile of depolarization along the axon since different parts of the membrane are at different stages of action potential generation (Figure 4). A region of membrane just in front of the action potential has not yet reached threshold, and a region behind is still depolarized from the falling edge of the action potential. The different states of these regions of membrane creates a fundamental asymmetry in the state of the ion channels in the regions flanking the location of the propagating action potential: Na+ channels are fully available in the region ahead of the action potential (yellow in Figure 4); Na+ channels far behind the action potential are also closed and hence ready to open (also yellow in Figure 4); Na+ channels in the region immediately trailing the action potential will be in the inactivated state (green in Figure 4), and hence unavailable for producing a subsequent action potential — thus this region of membrane is briefly refractory.
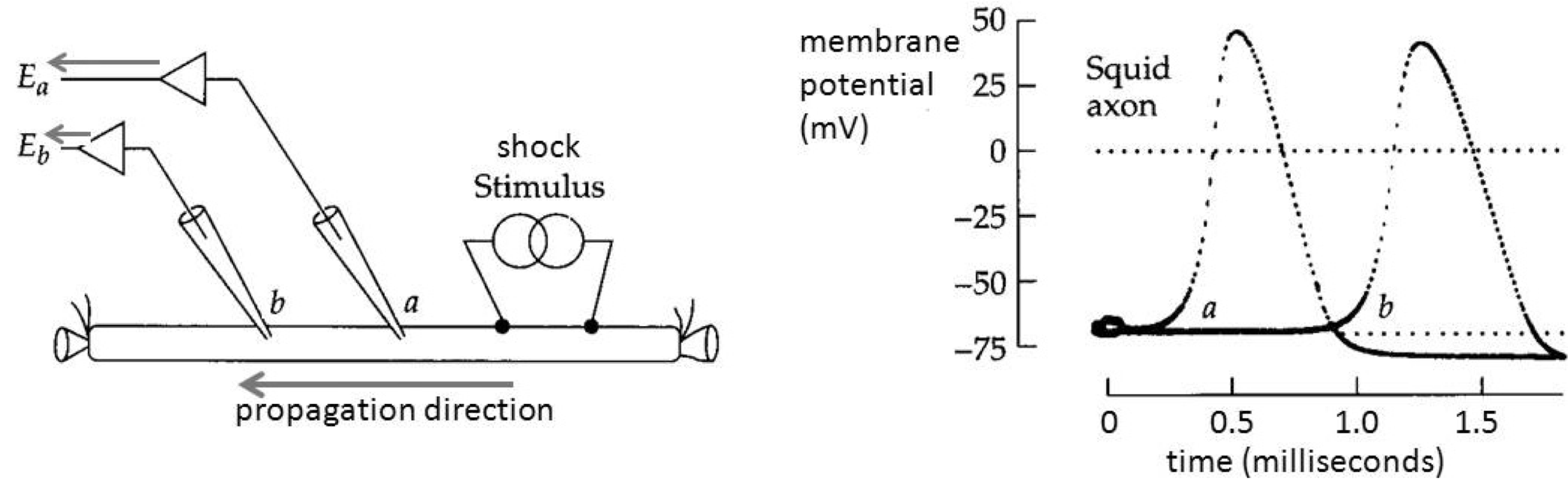
Depolarization of the membrane at the leading edge of the action potential opens Na+ channels (purple in Figure 4), and once a sufficient number have opened, the Hodgkin cycle is engaged and the action potential is regenerated. This sequential process causes the action potential to move down the axon. Propagation in the opposite direction is prevented due to the region of refractoriness (i.e. the green region of inactive Na+ channels) in the wake of the action potential. Thus, the directionality of propagation (from the soma to the axon terminal) down the axon is generated by the biophysical properties of Na+ channels (Action Potential, Threshold, Refractory Period Chapter, Figure 4) and the site of initiation at the axon hillock, not some intrinsic directionality of the axon itself.
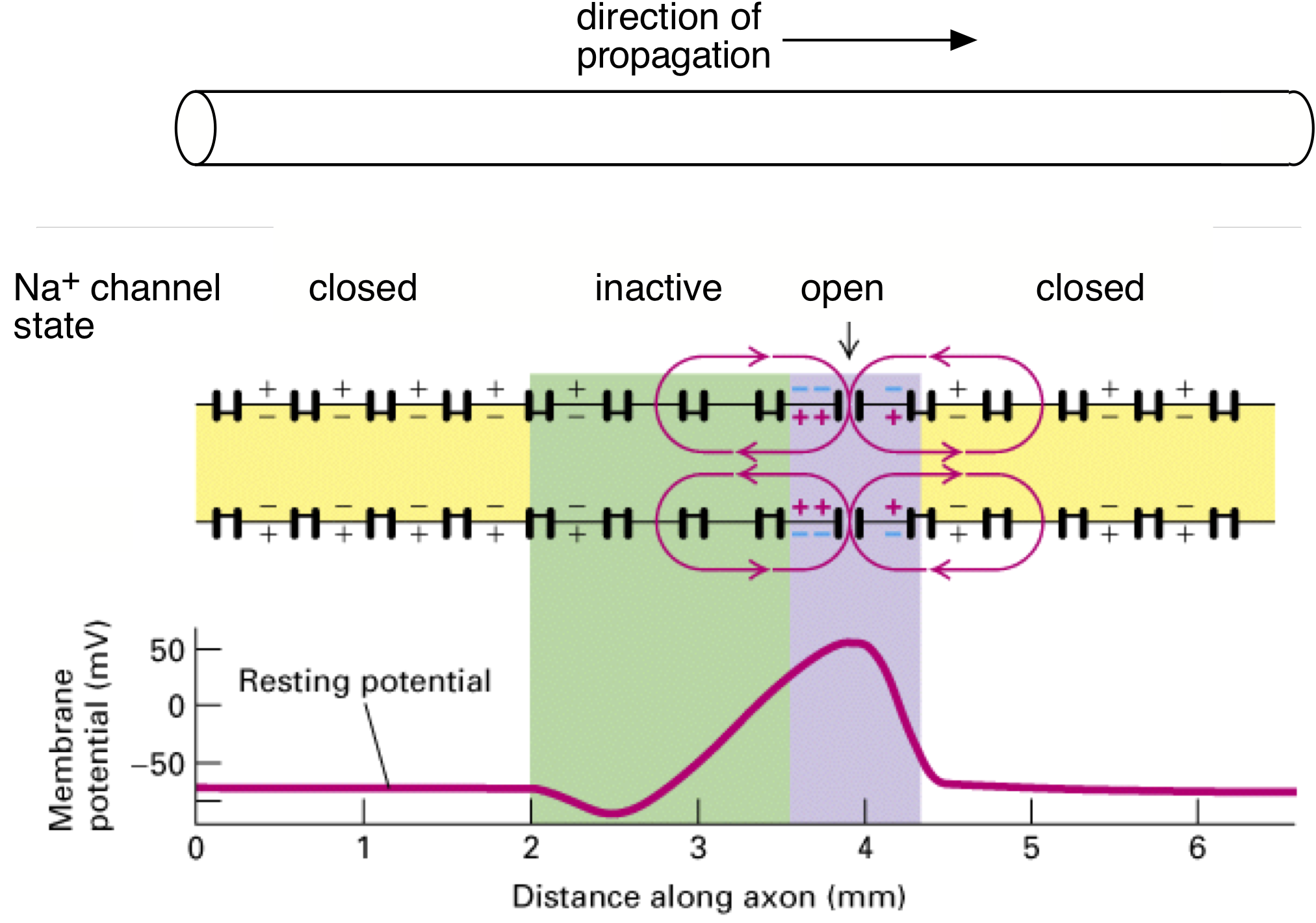
A simple exercise to check your understanding
The basic mechanism for propagation described above turns out to be much to slow to explain the speed of signaling in situations such as spinal reflexes. Two factors control the speed of propagation. One is the axon diameter: larger diameter axons have higher internal conductance and higher propagation speeds. Another key factor is the capacitance of the axon membrane.
What is capacitance and why does it slow action potential propagation? You have already encountered capacitance in the discussion of membranes (Membrane Potentials Chapter), but we review it here because it is a key factor controlling action potential propagation. For the membrane voltage to change, the electrical charge on the membrane must change. The relationship between charge and voltage is determined by the membrane capacitance: the greater the membrane capacitance, the more charge is required for a given voltage change (this is quantified as Q = CV, where Q is the charge on the membrane, C the capacitance and V is the membrane voltage). The charge comes from the current flowing into the cell (current is just charge per unit time). For a given amount of current, it takes longer to accumulate the larger charge required to change the voltage of a membrane with high capacitance than one with low capacitance. Think of a capacitor like a sponge in a cup. As you pour water into the cup, the sponge initially soaks it up. Only when the sponge is saturated does the water level in the cup rise. With a larger sponge, it will take more time to reach this point. Similarly, membrane capacitance “soaks up” incoming charge and slows changes in voltage.
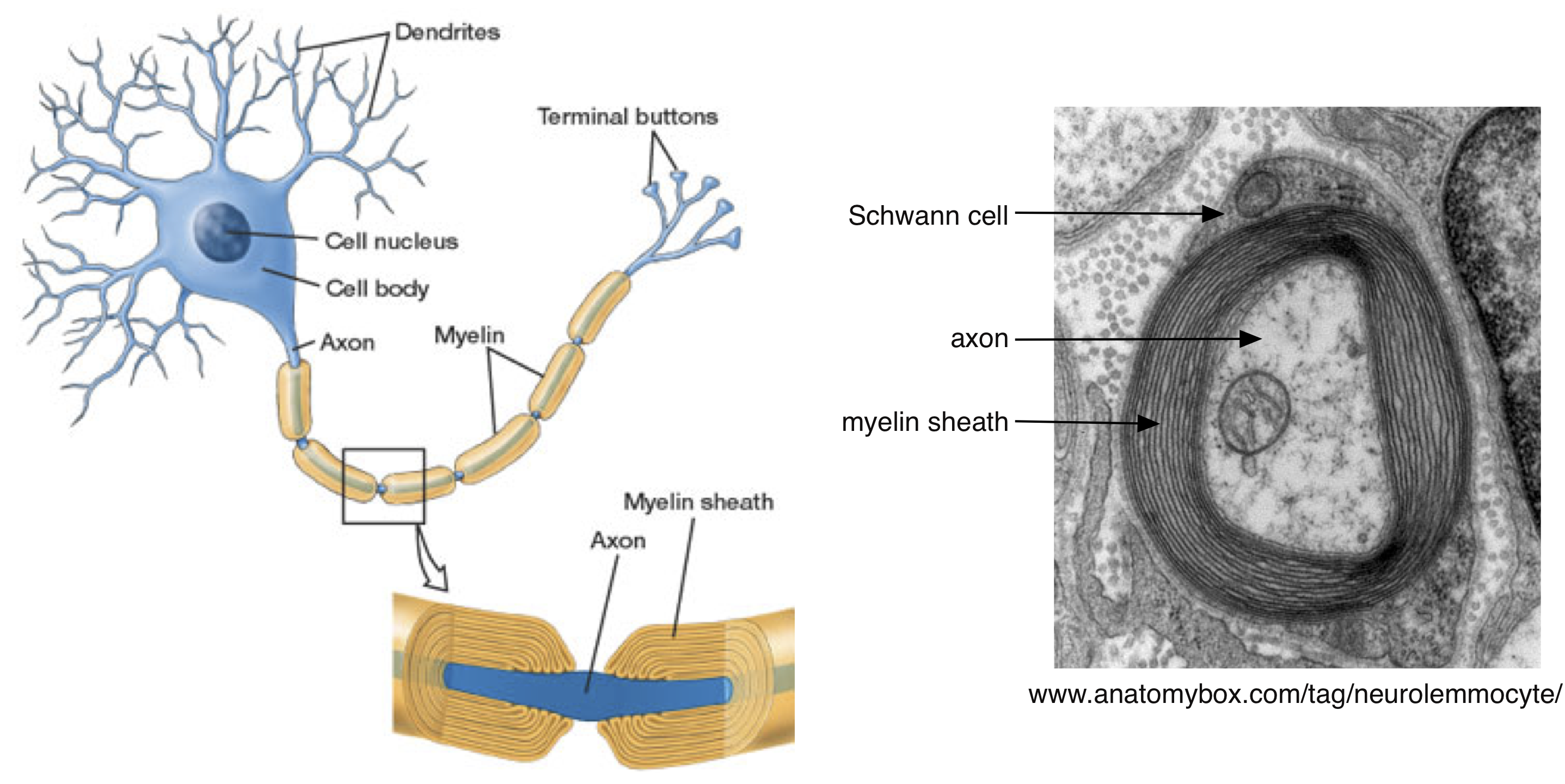
The need to charge the membrane capacitance poses a substantial limitation on the speed of action potential propagation. The solution that biology has reached to this problem is to wrap many axons — particularly those involved in long distance and rapid signal transmission — in myelin (Figure 5). Myelin is produced by glial cells (Schwann cells in the peripheral nervous system and oligodendrocytes in the central nervous system). Myelin is an electrical insulator — meaning that, like the cell membrane, charged ions cannot flow through it. The membrane capacitance is inversely proportional to the distance between the inner and outer membrane surfaces, and effectively thickening the membrane by wrapping it in myelin will decrease the capacitance. Myelin can decrease the capacitance by a factor of up to 1000. That means 1000 times less charge is needed to change the voltage!
Aside #1: The myelin sheath is compromised in diseases such as multiple sclerosis and Guillain-Barré, which can slow action potential propagation or cause it to fail.
In the absence of myelin, action potentials propagate by successively depolarizing each section of an axon, as in Figure 4. In myelinated axons, action potentials propagate by jumping between specific regions of the axons that lack myelin. These regions are called nodes of Ranvier (Figure 6), and Na+ channels are restricted to these nodes. Thus, the action potential is generated at one node and then propagates without being regenerated between the nodes. This is called “saltatory” conduction. The myelin sheath decreases the loss of current during propagation (because it decreases the membrane capacitance) between nodes and thus ensures that the depolarization at one node will be effective in depolarizing the next node and opening Na+ channels. Action potential propagation in myelinated axons can be more than 100 times faster than that in unmyelinated axons. Generation of the action potential at a node of Ranvier follows all the rules we discussed in the Action Potential, Threshold, Refractory Period Chapter and above (e.g. you can think of Figure 4 as referring to the status of Na+ channels at nodes ahead of and behind the action potential).
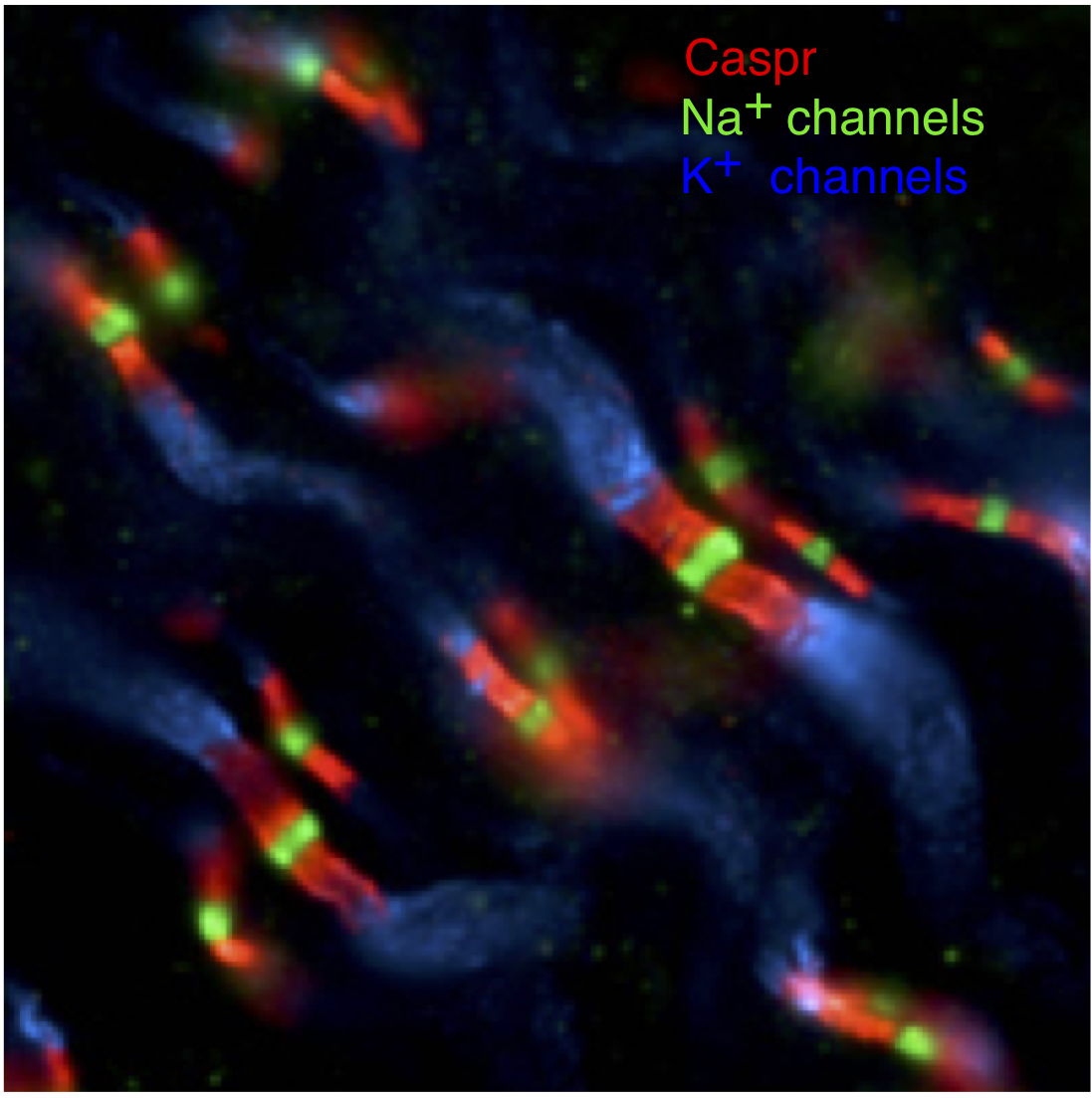
Some exercises to check your understanding
Practice Questions
Feedback: