3 Sensory transduction
Learning Objective 3: To compare the mechanisms of sensory transduction in different types of sensory receptors
All of the tissues in our body are endowed with sensory receptors with the ironic exception of the brain. In the following section, the mechanisms by which several different types of sensory receptors transform a physical stimulus into an electrical signal (i.e., sensory transduction) will be compared.
Light Receptors (Vision)
The principal light receptors (i.e., photoreceptors) in humans, rods and cones, are located in a thin layer of tissue lining the back of the eye called the retina. The mechanisms by which the photoreceptors convert light energy to an electrical signal are shown in the figure below. In the absence of a light stimulus, a ‘dark current’ flows through the membrane of the receptors carried by an influx of Na+ ions in the outer segment, and an efflux of K+ ions through the membranes of the inner segments. This current depolarizes the receptor membrane in the dark to about -40 mV. At this membrane potential, Ca2+ ions flow into the synaptic terminals of the photoreceptors resulting in the release of neurotransmitter. When light enters the eye through the pupil and impinges on the retina, it activates as a complex G-protein coupled receptor protein (called a photopigment) embedded on membranous disks within the outer segment of the photoreceptor. The photopigments are composed of a transmembrane opsin moiety and retinal, a chromophore. Absorption of light by the chromophore activates the opsin and then transducin, a G-protein, resulting in a decrease in the concentration of cGMP through phosphodiesterase (PDE) in the cytosol. The decrease in cGMP concentration in turns allows some of the Na+ channels to close, reducing the dark current, hyperpolarizing the receptor, reducing the influx Ca2+ in the synaptic terminals and consequently deceasing the release of neurotransmitter. This sequence of events is referred to as the phototransduction cascade. The process is terminated by the phosphorylation of the photopigment by a kinase and the binding of another protein called arrestin.
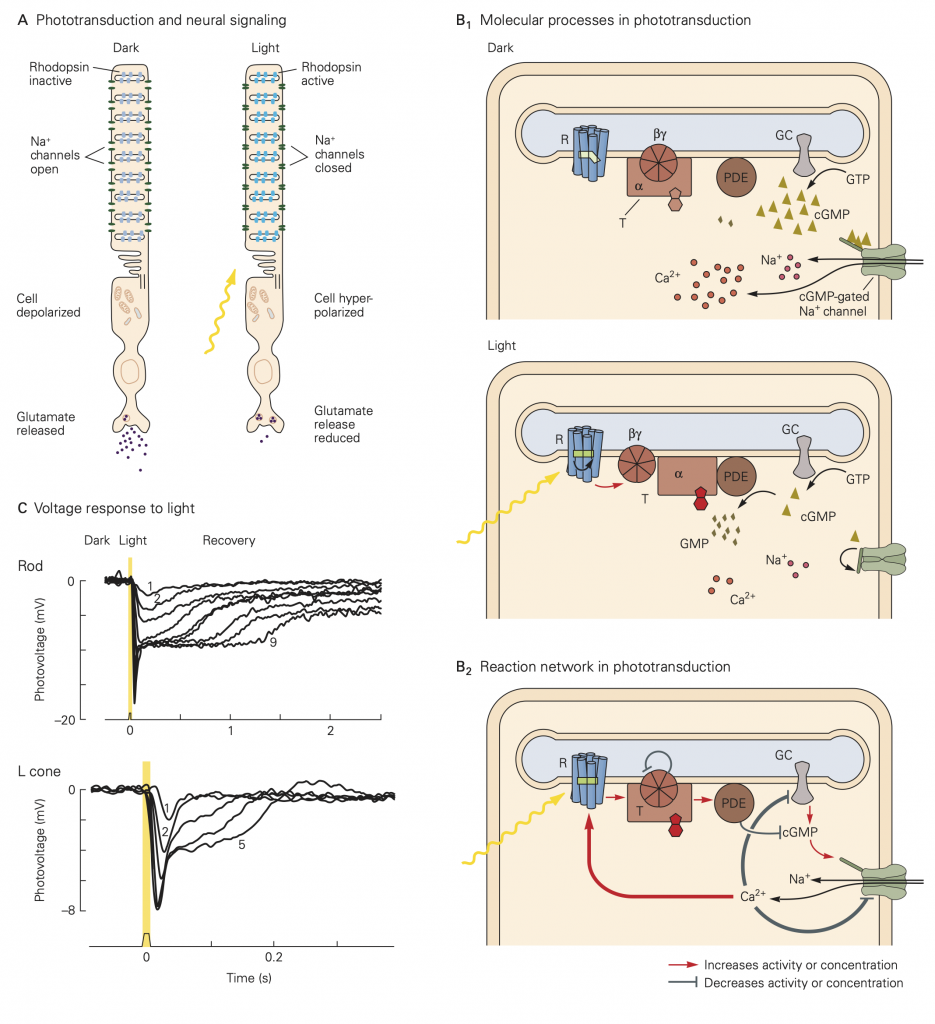
A. The rod cell responds to light. Rhodopsin molecules in the outer-segment discs absorb photons, which leads to the closure of cGMP-gated channels in the plasma membrane. This channel closure hyperpolarizes the membrane and reduces the rate of release of the neurotransmitter glutamate.
B. 1. Cyclic GMP (cyclic guanosine 3’-5’ monophosphate) is produced by a guanylate cyclase (GC) and hydrolyzed by a phosphodiesterase (PDE). In the dark the phosphodiesterase activity is low, the cGMP concentration is high, and the cGMP-gated channels are open, allowing the influx of Na+ and Ca2+. In the light rhodopsin (R) is excited by absorption of a photon, then activates transducin (T), which in turn activates the phosphodiesterase; the cGMP level drops, the membrane channels close, and less Na+ and Ca2+ enter the cell. The transduction enzymes are all located in the internal membrane discs, and the soluble ligand cGMP serves as a messenger to the plasma membrane. 2. Calcium ions have a negative feedback role in the reaction cascade in phototransduction. Stimulation of the network by light leads to the closure of the cGMP-gated channels. This causes a drop in the intracellular concentration of Ca2+. Because Ca2+ modulates the function of at least three components of the cascade—rhodopsin, guanylyl cyclase, and the cGMP-gated channel—the drop in Ca2+ counteracts the excitation caused by light.
C. Voltage response of a primate rod and cone to brief flashes of light of increasing intensity. Higher numbers on the traces indicate greater intensities of illumination (not all traces are labeled). For dim flashes the response amplitude increases linearly with intensity. At high intensities the receptor saturates and remains hyperpolarized steadily for some time after the flash; this leads to the afterimages that we perceive after a bright flash. Note that the response peaks earlier for brighter flashes and that cones respond faster than rods.
Chemoreceptors (Smell and Taste)
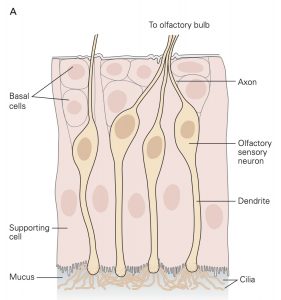
Sensory receptors that are designed to detect the presence of chemicals in the air we breathe, the substances we ingest and the fluids that circulate through our tissues are called chemoreceptors. The most familiar of these are olfactory sensory neurons (OSNs) embedded in the olfactory mucosa and the taste receptor cells found in the taste buds on the tongue and elsewhere in the oral cavity. The OSNs are endowed with olfactory cilia that extend into the mucosa of the oral cavity and interact with airborne odorant molecules. The olfactory cilia of each OSN express only one of the approximately 400 different olfactory receptor G-proteins such that each OSN is activated by a restricted set of odorant molecules. The olfactory sensory transduction cascade is quite similar to the phototransduction cascade except that the G-protein interacts with cyclase to increase cAMP rather than cGMP. The resultant depolarizing receptor potential leads to the generation of action potentials along the axon of the OSN. As is the case in phototransduction, the OSN receptor potential lasts until the olfactory receptor protein is phosphorylated by a kinase and bound to an arrestin-like protein so that the cAMP that was produced is hydrolyzed by PDE.
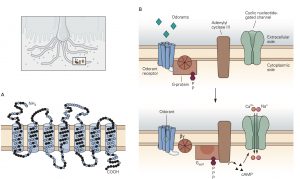
There are several different types of taste cells found in individual taste buds and three different types of sensory transduction mechanisms. Tastant molecules contained in ingested materials enter the taste buds through a taste pore on the surface of the tongue and other oral cavity structures and interact with taste receptors located on the microvilli of the taste cells. Full elucidation of the different transduction mechanisms underlying taste remains elusive, but the following descriptions are reasonable secure. Sweet, bitter and savory (umami) molecules bind to G-protein coupled taste receptors called, gustducins, leading to a depolarizing receptor potential in the taste that increases the release of neurotransmitter from the distal synaptic terminals. Sour, acidic substances and CO2 produce H+ ions in solutions that bind to sour taste receptor proteins and also flow through non-specific cation channels in the microvilli of sour taste cells. Finally, edible salts dissociate in liquid and the Na+ ions enter salty taste cells through ion channels in their microvilli leading to depolarization.
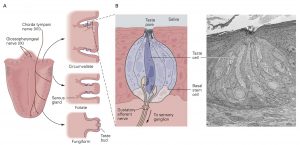
A. The three types of papillae—circumvallate, foliate, and fungi- form—differ in morphology and location on the tongue and are differentially innervated by the chorda tympani and glossopha- ryngeal nerves.
B. Each taste bud contains 50 to 150 elongated taste receptor cells, as well as supporting cells and a small population of basal stem cells. The taste cell extends microvilli into the taste pore, allowing it to detect tastants dissolved in saliva. At its basal end the taste cell contacts gustatory sensory neurons that transmit stimulus signals to the brain. The scanning electron micrograph shows a taste bud in a foliate papilla in a rabbit.
Mechanoreceptors (Touch and Proprioception)
Virtually of the tissues in the body are endowed with some type of mechanoreceptor designed to detect physical displacement, i.e., movement. For example, blood vessels are innervated by baroreceptors that respond to stretch of the vessel wall and thereby signal blood pressure to the CNS. Skeletal muscles contain two types of proprioceptors, called muscle spindles and Golgi tendon organs, which transduce muscle length and muscle force, respectively, into electrical signals that permit the CNS to precisely control movement. Common to all mechanoreceptors are membrane receptor proteins that form ion channels whose gating depends mechanical forces exerted on the channel protein. For example, applying stretch to a muscle also stretches the muscle spindles that reside within the muscle. This causes the stretch-sensitive channels in the membrane of the muscle spindle sensory receptors to open allowing Na+ ions to flow into the sensory neuron which in turn results in a depolarizing receptor potential. Relaxing the muscle stretch, removes the force on the mechanosensitive channels on the muscle spindle sensory receptors and the membrane of the sensory neuron returns to its resting level.
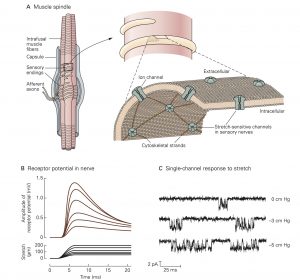
A. The muscle spindle is located within skeletal muscle and is excited by stretch of the muscle. It consists of a bundle of thin (intrafusal) muscle fibers entwined by a pair of sensory axons, and is also innervated by several motor axons (not shown) that produce contraction of the intrafusal muscle fibers. Stretch- sensitive ion channels in the sensory nerve terminals are linked to the cytoskeleton by the protein spectrin.
B. The depolarizing receptor potential recorded in a group Ia fiber innervating the muscle spindle (upper record) is proportional to both the velocity and amplitude of muscle stretch parallel to the myofilaments (lower record). When stretch is maintained at a fixed length, the receptor potential decays to a lower value.
C. Patch clamp recordings of a single stretch-sensitive channel in myocytes. Pressure is applied to the receptor cell membrane by suction. At rest (top record) the channel opens sporadically for short time intervals. As the pressure applied to the mem- brane increases (lower records) the channel opens more often and remains in the open state longer. This allows more current to flow into the receptor cell, resulting in higher levels of depolarization.
Skin is richly endowed with a host of different types of mechanoreceptors; each specialized to detect a particular form of mechanical stimulation. Also found in skin and many other tissues are nociceptors that are designed to detect noxious stimuli like extreme pressure, extreme temperature and dangerously high levels of chemical substances.
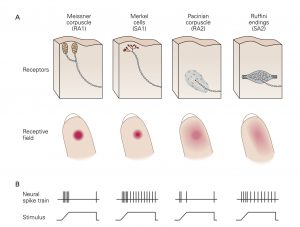
A. The superficial and deep layers of the glabrous (hairless) skin of the hand each contain distinct types of mechanoreceptors. The superficial layers contain small receptor cells: Meissner corpuscles and Merkel cells. The sensory nerve fibers innervating these receptors have branching terminals such that each fiber innervates multiple receptors of one type. The deep layers of the skin and subcutaneous tissue contain large receptors: Pacinian corpuscles and Ruffini endings. Each of these receptors. is innervated by a single nerve fiber, and each fiber innervates only one receptor. The receptive field of a mechanoreceptor reflects the location and distribution of its terminals in the skin. Touch receptors in the superficial layers of the skin have smaller receptive fields than those in the deep layers. (RA1, rapidly adapting type 1; RA2, rapidly adapting type 2; SA1, slowly adapting type 1; SA2, slowly adapting type 2.)
B. The nerve fibers innervating each type of mechanoreceptor respond differently when activated. The spike trains show responses of each type of nerve when its receptor is activated by constant pressure against the skin. The RA type fibers that innervate Meissner and Pacinian corpuscles adapt rapidly to constant stimulation while the SA type nerves that innervate Merkel cells and Ruffini endings adapt slowly.
Hair Cell Receptors (Hearing and Balance)
A highly specialized form of mechanoreceptor called the hair cell is found in the four different sense organs that reside within the bony labyrinth (i.e., cochlea, semicircular canals, utricle and saccule). The hair cells in the cochlea transduce sound waves that impinge on the tympanic membrane into changes in membrane potential and the hair cells in the semicircular canals, utricle and saccule are activated by movements of the head and body to help maintain object fixation in the visual field and balance.
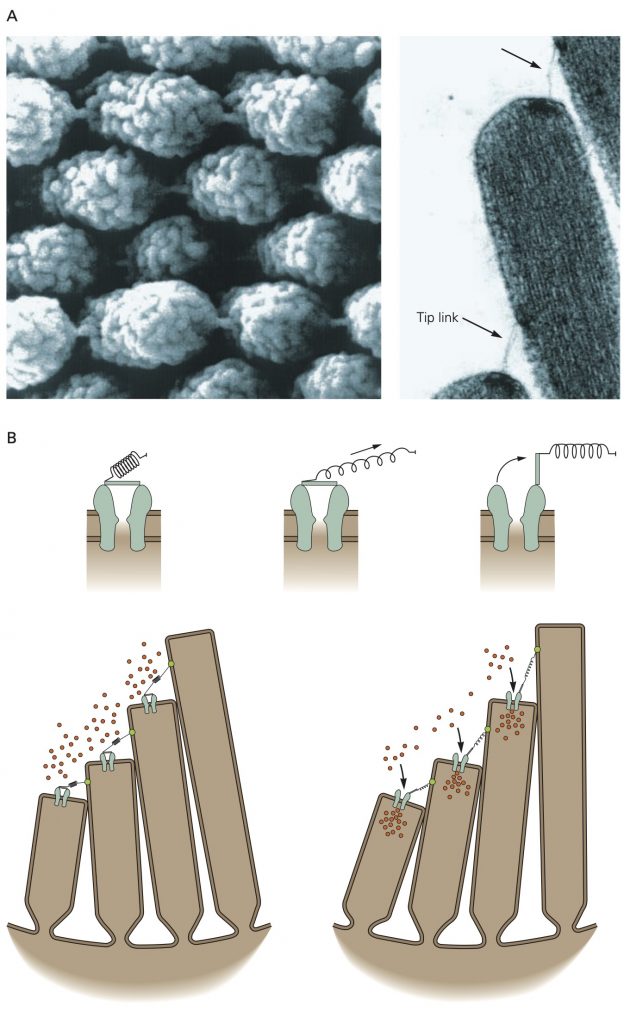
A. A tip link connects each stereocilium to the side of the longest adjacent stere- ocilium, as seen in a scanning electron micrograph (left) and a transmission electron micrograph (right) of a hair bundle’s top surface. Each tip link is only 3 nm in diameter. The links appear stouter in the illustration on the left because of metallic coating during specimen preparation.
B.Top: Ion flux through the channel that underlies mechanoelectrical transduction in hair cells is regulated by a molecular gate. The opening and closing of the gate are controlled by the tension in an elastic element, the gating spring, that senses hair-bundle displacement.
Bottom: When the hair bundle is at rest each transduction channel clatters between closed and open states, spending most of its time shut. Displacement of the bundle in the positive direction increases the tension in the gating spring, here assumed to be in part a tip link, attached to each channel’s molecular gate. The enhanced tension promotes channel opening and the influx of cations, thereby producing a depolarizing receptor potential.
Hair cells reside in an unusual extracellular milieu: the apical end that include the stereocilia that give the hair cells their name projects into endolymph which has an ionic composition similar cytosol (i.e., high K+, low Na+), while the remainder of the cell is bathed in perilymph with an ionic concentration similar to other extracellular fluids (high Na+, low K+). Pump molecules in the adjacent epithelia cells maintain the disparate ionic concentrations in these two compartments of the hair cells. K+ ions flow through non-selective cation channels located in the tips of the stereocilia, some of which are open at rest. Displacement of the stereocilia either stretches or relaxes molecular linkages between adjacent stereocilia that opens or closes the K+ channels, respectively. Opening more K+ channels leads to a depolarizing receptor potential whereas closing the K+ channels leads to a hyperpolarizing receptor potential. Depolarizing receptor potentials activate voltage-gated Ca2+ channels in the hair cell that results in more neurotransmitter release. With hyperpolarizing receptor potentials, the Ca2+ channels remain closed and the amount of neurotransmitter released is decreased.