2 Membrane Transport Proteins
Introduction
The intracellular and extracellular fluids are water based, so most substances dissolved in the body’s fluids are hydrophilic. The hydrophobic nature of the plasma membrane (cell membrane) creates a barrier that prevents the diffusion of most hydrophilic substances. Exceptions are small molecules such as gases like nitric oxide (NO) and carbon dioxide (CO2), and nonpolar (hydrophobic) substances such as steroid hormones and fatty acids.
Because of the barrier that the plasma membrane presents, transport of most substances depends upon transmembrane proteins, a process known as mediated transport. Below is a summary of the different types of transport processes and proteins.
Our focus is on the proteins that play a key role in epithelial transport. Ion channels are reviewed here, but will be discussed much more in the lecture portion of the class.
Channels
Channels are large proteins in which multiple subunits are arranged in a cluster so as to form a water-filled pore that passes through the membrane. Each subunit consists of multiple transmembrane domains.
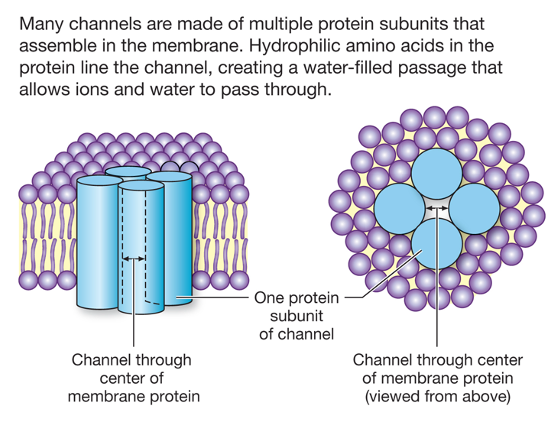
Most of the channels that we will consider are ion channels. Another important type of channel protein is an aquaporin. Aquaporins are channels that allow water to move rapidly across cell membranes via osmosis. Osmosis is the flow of water across a water-permeable membrane toward a region of higher solute concentration. We will discuss aquaporins when we discuss regulation of extracellular fluid osmolarity in the section of the course that deals with kidney physiology.
Movement through an open ion channel does not require ATP energy, so it is a passive process. There is also no specific binding of ions to the channel protein. The two factors that affect the flow of ions through an open ion channel are the membrane potential and the concentration gradient. Note that when ions move through a channel across a membrane, this changes the membrane potential (depolarization or hyperpolarization). Changes in membrane potential are used to code information, particularly in the nervous system.
For any ion channel, there are two important properties to consider: selectivity and gating. Selectivity refers to which ion (Na+, K+, Ca++, or Cl–) is allowed to travel through the channel. Most ion channels are specific for one particular ion. Gating refers to what opens or closes a channel. Some channels are opened by changes in membrane potential (voltage-gated) such as the voltage-gated Na+ channel involved in electrical excitability in neurons. Some channels are ligand-gated and are opened when a regulatory molecule binds to them, such as the nicotinic acetylcholine receptor, involved in synaptic transmission at the neuromuscular junction. Ion channels in sensory neurons may be mechanically-gated, temperature-gated, or gated by factors that cause tissue damage (pain-sensitive neurons).
Facilitated Diffusion
Facilitated diffusion is transport involving a carrier protein that has a specific binding site for the transported substance. An example is the transport of glucose into cells (glucose uptake) following a meal. The transport protein, known as the glucose transporter (or GLUT), has a specific binding site for glucose.
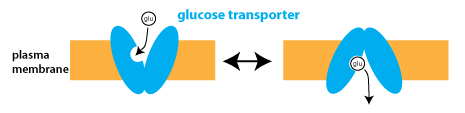
The binding of glucose changes the conformation of the glucose transporter, which can exist in two different conformations that expose the binding site to either the extracellular fluid or the cytosol (intracellular fluid).
The concentration gradient for glucose determines the rate and direction of transport. Facilitated diffusion is a passive process, meaning that it does not require ATP hydrolysis. With glucose uptake, glucose is transported from the extracellular fluid into the cytosol, where cells rapidly phosphorylate it to create glucose-6-phosphate, preventing glucose from building up or leaving the cell.
Facilitated diffusion and other processes that depend on membrane transport proteins can be regulated by controlling the number of transport proteins present in the membrane. For instance, glucose uptake is regulated by the hormone insulin. At low concentrations of insulin, few glucose transporters are on the plasma membrane. Insulin stimulates glucose uptake by causing vesicles containing glucose transporters to fuse with the plasma membrane, as shown in the figure below.
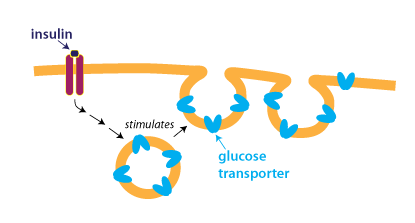
Active Transport
Active transport describes the process whereby the transport of specific substances is coupled to ATP hydrolysis. In primary active transport, the carrier protein hydrolyzes ATP in order to change conformation and transport substances across the membrane. Because the energy for transport is derived from ATP hydrolysis, these transporters effectively move substances in one direction, and can transport substances against a concentration gradient.
Primary active transporters are generally known as ATPases because they hydrolyze ATP. The most widespread and physiologically important active transporter in cells is the Na+/K+-ATPase, or sodium-potassium pump. This protein moves three Na+ ions out of the cell and two K+ ions into the cell with each cycle of ATP hydrolysis. (Although the schematic below doesn’t depict it as such, the Na+/K+-ATPase is a carrier protein with two conformations that alternately expose binding sites for Na+ and K+ ions to the intracellular and extracellular fluid, similar to the GLUT protein).
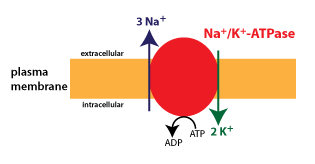
The Na+/K+-ATPase is expressed in all cells, and is responsible for generating the typical Na+ and K+ gradients found across the cell membrane.
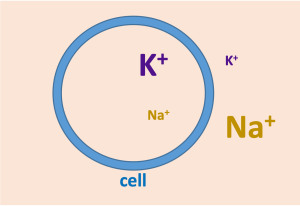
These ionic gradients underlie the membrane potential and electrical excitability in neurons and muscles. As well, the Na+ gradient is used to power coupled transport of glucose and many other substances, as discussed below. It is estimated that in a body at rest, the activity of the Na+/K+-ATPase consumes about a third of all ATP.
Other important active transporters include Ca++-ATPases and the H+/K+-ATPase. Ca++-ATPases keep the Ca++concentration low in the cytosol. One type of Ca++-ATPase is found in the plasma membrane; another is found in the membrane of the endoplasmic reticulum and the sarcoplasmic reticulum of muscle cells. The H+/K+-ATPase or “proton pump” is responsible for acid secretion in the stomach.
Secondary Active Transport
Secondary active transport (or coupled transport) utilizes the energy inherent in the Na+ gradient to transport substances. Coupled transport is similar to facilitated diffusion in that it involves specific binding, however in this case, two substancesare required to bind in order for transport to occur. As a consequence, the free energy driving the transport is the sum of the free energies for transport of both substances. If the transported substances move in the same direction across the membrane, it is called cotransport (or symport); if they move in the opposite direction, it is called countertransport (or antiport).
The transport of glucose across the apical plasma membrane of epithelial cells in the small intestine is an example of cotransport. This is the first step in the absorption of glucose in the digestive tract. The transport protein is known as the sodium-glucose cotransporter (or SGLT).
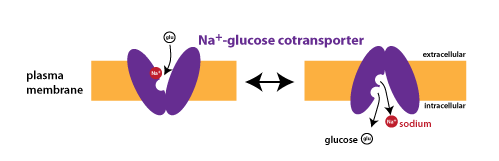
Immediately after eating a lot of carbohydrates, the concentration gradient of glucose will favor transport into cells, but this concentration gradient disappears as more and more glucose is absorbed. However, there is always a steep concentration gradient favoring the movement of Na+ into cells, because the concentration of Na+ inside of cells is kept very low through the constant action of the sodium-potassium pump (Na+/K+-ATPase, see above). Because transport is coupled, the Na+ concentration gradient can power the movement of glucose uphill against its concentration gradient. Unlike facilitated diffusion, coupled transport is an active process since ATP hydrolysis is required to establish the Na+ gradient.
Carrier Proteins Show Saturation Kinetics
Because they both involve specific binding, facilitated diffusion and coupled transport show saturation. Transport depends upon a limited number of transport proteins in the membrane, each of which must bind with the transported substance for a given period of time.
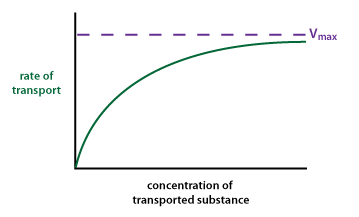
ABC Transporters
ABC transporters are a family of transport proteins that depend upon ATP binding for transport. ABC stands for ATP-Binding Cassette. ABC proteins have a particular molecular structure that includes two nucleotide binding domains where ATP binds.
Most ABC proteins act as active transport pumps. An example is the protein P-glycoprotein 1 (also known as MDR 1 for multi drug resistance protein 1). The structure of this protein is depicted in the figure.
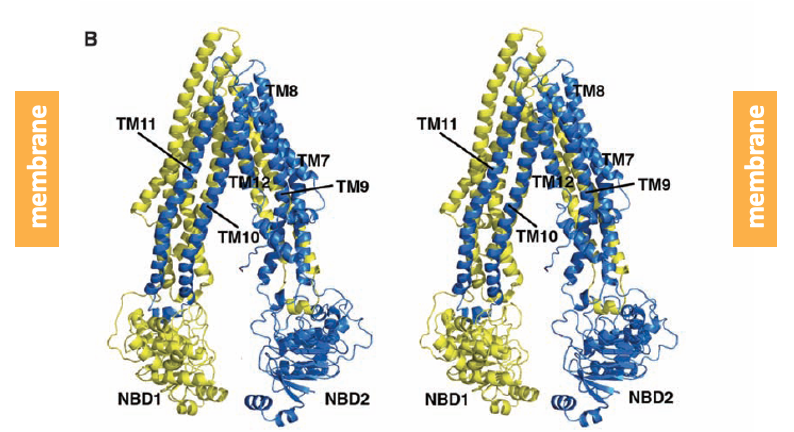
P-glycoprotein 1 contains two groups of alpha-helices that span the plasma membrane. On its intracellular face, the protein has two nucleotide binding domains (NBD) where ATP binds. ATP hydrolysis is coupled to the efflux of a broad spectrum of substrates. Up-regulation of the activity of P-glycoprotein 1 plays a role in the development of resistance to chemotherapy drugs.
A unique and physiologically important member of the ABC transporter family is the protein CFTR. CFTR stands for “cystic fibrosis transmembrane condcutance regulator”, an unwieldy name that I do not expect you to learn. CFTR is not a pump, rather it is a Cl– channel that is expressed by many epithelial cells.
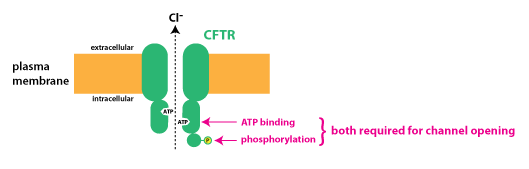
CFTR is the protein that is defective in the genetic disorder cystic fibrosis. Unlike most ABC transporter proteins that use the energy of ATP hydrolysis to pump substances across the membrane and out of cells, CFTR works as a ligand-gated ion channel that requires both ATP binding and phosphorylation by the enzyme protein kinase A in order to open.
CFTR plays a key role in the secretion of fluid across epithelia (see the next chapter on Epithelial Transport). In cystic fibrosis, CFTR channels are defective and/or absent. This leads to decreased fluid secretion and causes pathology in the lungs and digestive system.