18 Early-Phase Material Optimization for Reduced Embodied Carbon Impact
Nikhil Kumar Palugulla
1. Motivation for the problem
The construction industry is responsible for a significant portion of global carbon emissions, with embodied carbon from building materials being a major contributor. Addressing this issue at the early design phase presents a crucial opportunity to reduce the environmental impact of buildings. The motivation behind studying early-phase material optimization stems from the potential to make informed decisions that prioritize low-carbon solutions from the outset when the potential for impact is greatest. By integrating tools like “2050 Materials” and “One-Click LCA” into the conceptual design process, architects and designers can assess the embodied carbon footprint of various materials and structural systems, enabling them to explore and compare multiple design alternatives.
2. Purpose & Focus
The primary purpose of this research is to investigate how the integration of tools like “2050 Materials” and “One-Click LCA” into the early design process can reshape the architectural approach towards material selection and optimization. By providing data-driven insights into the carbon footprint of building materials, these tools empower architects to make informed decisions that prioritize low-carbon solutions from the conceptual stage. The focus is on understanding the implications of this carbon-conscious decision-making process and how it can lead to more sustainable and environmentally responsible designs. Additionally, the research aims to explore the potential benefits of early-phase material optimization, such as reduced environmental impact, improved regulatory compliance, and enhanced collaboration among stakeholders.
3. Facts related to the case
The Royal Institution of Chartered Surveyors (RICS) categorizes building elements for whole-life carbon assessments, mandating consideration of Substructure and Superstructure categories, while other categories can be optional. In assessing the entire lifecycle of a building, elements are first categorized, followed by calculating the lifecycle carbon emissions[1]. The lifecycle stages include Product stage (A1-A3), covering raw material extraction, transportation to the manufacturing plant, and manufacturing processes, termed ‘cradle to gate’; Construction stage (A4-A5), involving material transportation to the site and construction and installation processes, known as ‘cradle to practical completion’; In-use stage (B1-B7), encompassing embodied carbon from usage, maintenance, replacement, repair, and refurbishment, as well as operational carbon emissions from energy and water usage, labeled ‘cradle to end of life’; End of life stage (C1-C4), including demolition, waste transportation, processing, and disposal, referred to as ‘cradle to grave’; and Beyond the life cycle (D), which considers the potential for recycling and reusing materials[1].
![Figure 1: Whole Life Cycle Stages[1]](https://uw.pressbooks.pub/app/uploads/sites/795/2024/04/Screenshot-2024-05-28-101808-1024x616.png)
2050 Materials is a digital platform that provides a comprehensive database and tools to help architects, engineers, and contractors research, compare, and select sustainable building materials based on their environmental impacts, particularly embodied carbon emissions[2]. One-Click LCA is a life cycle metrics software that estimates the embodied carbon in building materials based only on the location, size, and type of building. Users can instantly create baseline buildings or concept phase options, as well as calculate an early-stage life cycle assessment[3].
The term embodied carbon encompasses greenhouse gases (GHGs) emitted during the extraction, manufacture, transportation, construction, replacement, and deconstruction of building materials, as well as end-of-life emissions. Embodied carbon in building materials typically accounts for between 39% and 80% of a building’s total carbon footprint. If assessed early in the design process, up to 80% of a building’s embodied carbon can be reduced[4]. Using carbon-neutral building materials yields significant environmental benefits, as these materials emit no net greenhouse gases throughout their life cycle. This aligns with the Paris Agreement’s goal of limiting global warming to 1.5° Celsius. Given that the building sector contributes 39% of global emissions, reducing embodied carbon in materials emerges as a practical mitigation strategy. Carbon-neutral materials further sustainability efforts by reversing environmental degradation and promoting clean, renewable energy sectors. Moreover, certain carbon-negative materials, such as biochar and hempcrete, actively remove and store carbon, functioning as carbon sinks. Innovative materials, like recycled metals and low-carbon bricks, not only decrease emissions but also diminish waste and pollution, thereby enhancing air quality and biodiversity[5].
![Figure 2: Carbon emission by building type and Element[6]](https://uw.pressbooks.pub/app/uploads/sites/795/2024/04/Screenshot-2024-05-28-003759-1024x453.png)
One of the primary ways these tools transform the design process is by allowing data-driven decision-making from the start. Akadiri et al. (2012) discovered that incorporating embodied carbon considerations into the design process can result in reductions of up to 40% in the total embodied carbon of a building[7]. This demonstrates how important early-phase material optimization can be for reducing a project’s environmental footprint. By giving architects access to detailed data on the embodied carbon, water footprint, and other sustainability metrics of various building materials, these tools enable them to make informed decisions that prioritize low-impact solutions from the start. Furthermore, early-phase material optimization tools enable an iterative design process, allowing architects to test multiple options and refine their decisions based on environmental impact assessments. Cabeza et al. (2013) found that the embodied energy of building materials varies significantly, with some materials having up to ten times more energy than others[8]. By allowing architects to compare the environmental impact of various material options during the conceptual design phase, these tools encourage a more holistic and sustainable approach to material selection.
![Figure 3: Opportunities to reduce embodied carbon across all project phases[9]](https://uw.pressbooks.pub/app/uploads/sites/795/2024/04/build-smart-1.png)
4. Data and Pattern
The data collected for this study includes both quantitative data and qualitative analysis:
-
- A carbon footprint assessment using the “2050 Materials” tool for a low-rise residential building focusing on stages A1-A3 (Product stage). The assessment demonstrated how the switching from traditional materials to sustainable alternatives reduced total carbon emissions and the interpretation of how this result changed the design process.
- Analyzing the Impact of Material Choices and Construction Methods on Embodied Carbon: A Case Study of 300 Gray’s Inn Road Building and Its Influence on Design Decision-making.
5. Patterns/theories found
5.1: A carbon footprint assessment using the “2050 Materials” tool for a low-rise residential building focusing on stages A1-A3(Product stage)
Overview: To conduct a carbon footprint assessment using the “2050 Materials” tool for a low-rise residential building, I start by selecting the building type and its size (e.g., low-rise or high-rise). This selection redirects us to a page where we can input and adjust the relevant data. Utilizing the building specification details provided in Table 1, I first input traditional materials(Figure 2) and then repeat the process using sustainable materials(Figure 3). This approach allows us to compare the carbon emissions of traditional versus sustainable materials specifically during stages A1-A3 (Product stage), which include raw material extraction, transportation to the manufacturing plant, and the manufacturing process.
Project goals: The project goal is to compare the environmental impact of traditional versus sustainable materials in a low-rise residential building during stages A1-A3 (Product stage) using the “2050 Materials” tool.
S.no | Building Specifications | |
1 | Number of People | 15 |
2 | Floor area | 750m2 |
3 | Expected building lifetime | 80 years |
4 | Estimated Value | Euro150,000 |
Table 1: Building Specifications
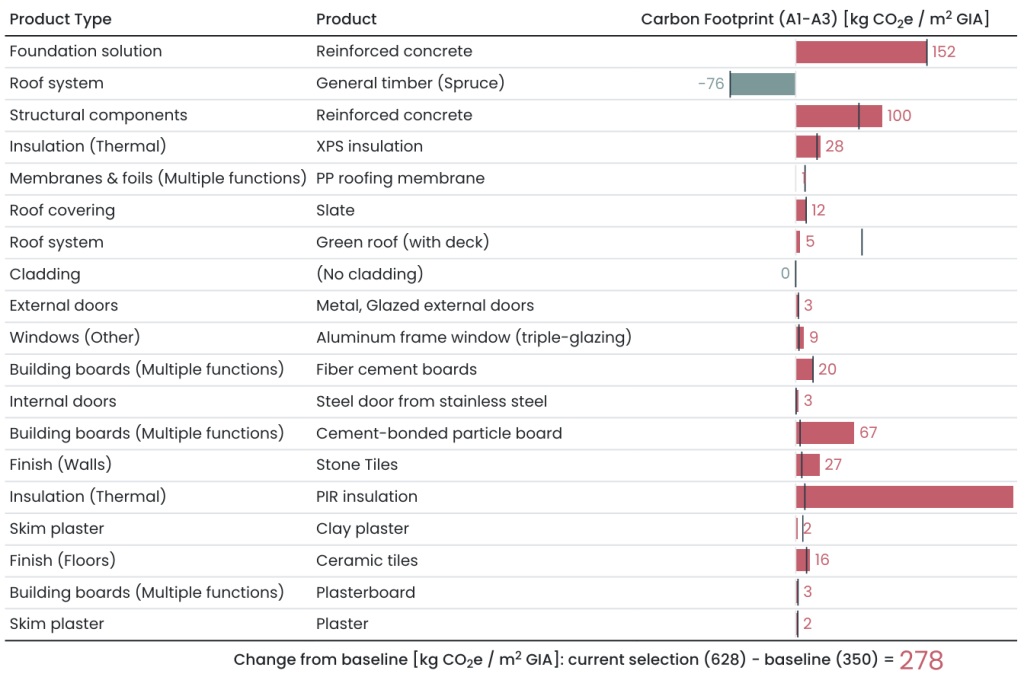
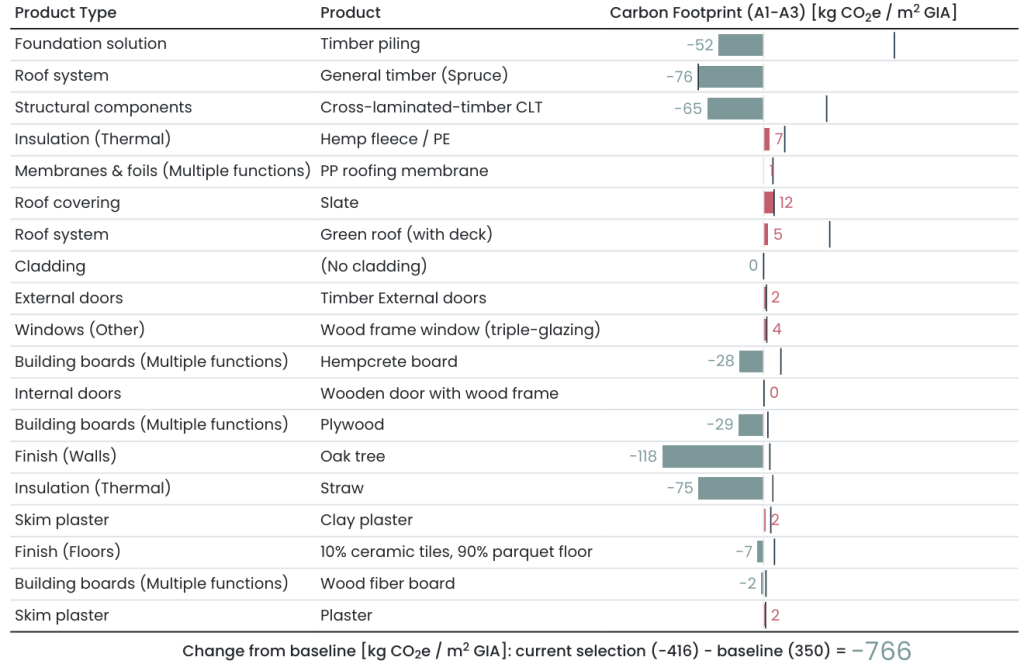
S.no | Type | Impact of using selected traditional materials on carbon emissions | Impact of switching from selected traditional materials to sustainable materials |
1 | CO2e (A1-A3), total | 471,000 kg CO2e | −312,000 kg CO2e |
2 | CO2e (A1-A3), per m per year | 7.8 kg CO2e / m2 / yr | −5.2 kg CO2e / m2 / yr |
3 | CO2e (A1-A3), per person per year | 393 kg CO2e / person / yr | −260.0 kg CO2e / person/yr |
4 | Freshwater used (A1-A3), total | 280,500 m3 | 2,250 m3 |
5 | Embodied carbon offset cost | £ 37,500 | £ −24,750 |
6 | Percentage of project value | 25% | −16.5% |
Table 4: Building Specific Impact Report
Key findings: Based on the results presented(Table 4), the key conclusion is that material selection plays a crucial role in determining the environmental impact of a building, particularly in terms of carbon footprint, warming potential, and water footprint. The option that incorporates sustainable and low-carbon materials, such as timber, cross-laminated timber (CLT), hemp insulation, and natural finishes, demonstrates significantly lower environmental impacts compared to the option that relies on conventional materials like reinforced concrete, XPS insulation, and ceramic tiles.
These findings have significant implications for the design process and the way architects approach their projects. Firstly, it highlights the importance of conducting thorough life cycle assessments (LCAs) and embodied carbon calculations during the early design stages. By quantifying the environmental impacts of different material choices, architects can make informed decisions that prioritize sustainability and minimize the carbon footprint of their projects.
Secondly, the results encourage architects to explore and embrace innovative low-carbon materials and construction techniques. The use of CLT, for instance, not only offers a sustainable alternative to conventional materials but also enables prefabrication and modular construction. Prefabricated CLT components can be manufactured off-site, reducing construction waste and emissions, and then transported and assembled on-site, potentially reducing construction time and costs. Moreover, the modular and relocatable nature of prefabricated CLT structures allows for greater flexibility and adaptability. Buildings constructed using CLT can be disassembled and relocated to different sites as needed, extending their lifespan and reducing the need for demolition and new construction. This aligns with the principles of circular economy and supports a more sustainable approach to the built environment.
Conclusion: The results emphasize the need for a paradigm shift in the design process, where environmental considerations and sustainable material choices are integrated from the outset. Architects play a pivotal role in driving this change by embracing innovative low-carbon materials, prefabrication techniques, and circular design principles. By doing so, they can create buildings that not only meet functional and aesthetic requirements but also minimize their environmental impact, contributing to a more sustainable and resilient built environment.
5.2: Analyzing the Impact of Material Choices and Construction Methods on Embodied Carbon focusing: A Case Study of 300 Gray’s Inn Road Building and Its Influence on Design Decision-making.
Overview: 300 Gray’s Inn Road, a 1970s office building 500 meters south of Kings Cross Station, is undergoing significant renovations. The existing 8-story structure, along with a 3-story block, is out of date, with an EPC rating of ‘D’. This rating, combined with upcoming EPC/MEES legislation, renders the building unlettable by 2027. Despite its deteriorating state, the building’s prime location in an economically active area creates an opportunity for redevelopment into a sustainable commercial landmark[10].
Project Goals: The primary objective is to retrofit 300 Gray’s Inn Road into an exemplary low-carbon building, with a target completion date of 2026. This transformation involves:
-
- Creating an entirely new façade while retaining 88% of the internal structure.
- Meeting and exceeding statutory sustainability requirements.
- Reducing embodied carbon through innovative design and material choices[10].
![Figure 4: Different stages of the building renovation project[10]](https://uw.pressbooks.pub/app/uploads/sites/795/2024/04/Screenshot-2024-05-28-131300-1024x714.png)
Sustainable Design Approach – Façade Design: The design of a new facade is critical to the project’s sustainability goals, as facades are significant contributors to embodied carbon in buildings. The project team, leveraging One Click LCA, focused on detailed assessments of facade components to identify carbon hotspots and explore sustainable alternatives[10].
One Click LCA Integration: Haptic utilized One Click LCA to support its sustainability objectives from the early design stages. This tool enabled:
-
- Detailed analysis of embodied carbon at the component level.
- Identification of carbon-intensive elements and potential sustainable substitutes.
- Transparent reporting and robust evaluation to guide design improvements[10].
Key Findings: The detailed analysis revealed that the typical bays (1A+1B) within the existing facade, constituting 40% of the development, have critical carbon-intensive elements.
![Figure 4: Types of the typical bays within the existing facade[10]](https://uw.pressbooks.pub/app/uploads/sites/795/2024/04/Screenshot-2024-05-28-131322-1024x770.png)
![Figure 5: Detail of typical bay(1A+1B)[10]](https://uw.pressbooks.pub/app/uploads/sites/795/2024/04/Screenshot-2024-05-28-131434-1024x761.png)
The top five contributors to A1-A5(Product and Construction stage) facade emissions in the typical bay(1A+1B) include GRC facade cladding, Aluminum frame windows, Rock wool insulation panels, Light gauge steel for facade infill, and Aluminum perforated sheets.
Utilizing One-Click LCA by examining alternative materials and design strategies, the team achieved significant reductions in embodied carbon. The new facade will be made up of glass fiber reinforced concrete (GRC), masonry, and some precast concrete elements. The project’s vision includes ensuring that the new facade is holistically high-performing, which means it has low embodied carbon while still providing good thermal performance, passive solar gain, and natural ventilation capabilities. The A1-A5 Upfront Embodied Carbon for Bay 1A was estimated at 82.3 kgCO2/m², 20% below the target[10].
Conclusion: The transformation of 300 Gray’s Inn Road demonstrates Haptic’s commitment to sustainable architecture. By incorporating One Click LCA into the early design phase, Haptic was able to achieve significant visibility and comparability of embodied carbon, allowing for informed decision-making and innovative design solutions. This project is part of the Haptic Green initiative, which aims to set a new standard for sustainable design and demonstrate the feasibility of low-carbon retrofits in urban environments[10].
6. Connection to the larger scheme of things
The data from these case studies showcases a significant shift in the construction and design paradigm towards sustainability and reduced carbon emissions. The integration of tools like “2050 Materials” and “One-Click LCA” has enabled architects and designers to make data-driven decisions that prioritize low-carbon solutions from the conceptual stage. This shift has several connections to the larger scheme of things:
One of the significant implications of these case studies is the tangible impact of reducing embodied carbon. By carefully selecting materials and construction methods, significant reductions in carbon emissions can be achieved across different building types and stages. This reduction is crucial in the context of global efforts to mitigate climate change, where the construction industry plays a substantial role due to its carbon-intensive nature.
Moreover, these case studies highlight the industry’s capacity for innovation and adaptation. The adoption of innovative materials like cross-laminated timber (CLT) and glass fiber reinforced concrete (GRC), coupled with sustainable design strategies, underscores a commitment to pushing the boundaries of sustainable construction practices. This not only benefits the environment but also contributes to the development of a more resilient and future-proof built environment.
Another key aspect is the emphasis on circular economy principles, such as modular construction and material reuse. These principles not only reduce waste and environmental impact but also promote more efficient use of resources throughout the building lifecycle. This shift towards circularity aligns with broader sustainability goals and fosters a more holistic approach to design and construction.
Furthermore, the collaborative nature of these tools promotes stakeholder engagement and transparency. Architects, engineers, contractors, and clients can work together more effectively, leveraging data-driven insights to align on sustainability objectives and make informed decisions. This collaborative approach is essential for driving meaningful change and ensuring that sustainability remains a core consideration in every project.
7. Conclusion
In conclusion, the integration of tools like “2050 Materials” and “One Click LCA” marks a significant shift in the design process within the construction industry. These tools bring data-driven decision-making to the forefront, enabling architects to prioritize sustainability and reduce environmental impact from the earliest conceptual stages. By providing insights into embodied carbon and encouraging the use of low-carbon materials, these tools not only contribute to meeting regulatory requirements and enhancing collaboration but also foster a culture of innovation and responsibility in architectural design. This transformation in the design process is crucial for creating a more sustainable built environment and aligns with global efforts to address climate change effectively.
8. References
[1] E. Savran, University of Westminster, College of Design, Creative and Digital Industries, and School of Architecture and Cities, “The impact of material selection and construction strategies on embodied carbon: A case study analysis of W3 King’s Cross,” thesis, Jul. 2022. [Online]. Available: https://hapticarchitects.com/wp-content/uploads/2023/08/The-Impact-of-Material-Selection-and-Construction-Strategies-on-Embodied-Carbon-A-Case-Study-Analysis-of-W3-Kings-Cross.pdf
[2] N. Makhoul, “Embodied Carbon Optimizer tool for Building Assemblies with 2050 Materials,” 2050 Materials – Simplifying Sustainable Material Selection, Mar. 04, 2024. https://2050-materials.com/blog/discover-embodied-carbon-optimizer-tool-for-building-assemblies-with-2050-materials/
[3] A. Ramachandran, “One click LCA & Autocase: tell the full carbon story of buildings,” One-Click LCA, Apr. 11, 2024. https://oneclicklca.com/en/resources/press-release/autocase-integration-carbon-buildings#:~:text=One%20Click%20LCA%20is%20a,calculate%20an%20early%2Dstage%20LCA
[4] “How to reduce embodied carbon in building design | Cove.Tool,” Apr. 30, 2024. https://cove.tools/blog/carbon-reducing-embodied-carbon-early-design
[5] J. L, “Carbon negative building materials,” Carbon Credits, Mar. 07, 2023. https://carboncredits.com/carbon-negative-building-materials/
[6] SGH, “Embodied Carbon in Structures: Reducing Environmental Impact in the AEC Industry – SGH,” SGH, Jan. 08, 2024. https://www.sgh.com/insight/embodied-carbon-in-structures/
[7] “Design of a Sustainable Building: A conceptual framework for implementing sustainability in the building sector,” Researchgate, Apr. 2012, [Online]. Available: https://www.researchgate.net/publication/224893604_Design_of_A_Sustainable_Building_A_Conceptual_Framework_for_Implementing_Sustainability_in_the_Building_Sector
[8] L. F. Cabeza, C. Barreneche, L. Miró, J. M. Morera, E. Bartolí, and A. I. Fernández, “Low carbon and low embodied energy materials in buildings: A review,” Renewable & Sustainable Energy Reviews, vol. 23, pp. 536–542, Jul. 2013, doi: 10.1016/j.rser.2013.03.017
[9] “DESIGN GUIDANCE FOR REDUCING EMBODIED CARBON IN STRUCTURAL SYSTEMS – SE2050.” https://se2050.org/resources-overview/structural-materials/lean-design-guidance/
[10] J. Moore, “Sustainable Architecture at Haptic,” Oneclicklca, May 27, 2024. https://oneclicklca.com/en/resources/case-studies/sustainable-architecture-at-haptic
Media Attributions
- Figure 1: Whole Life Cycle Stages[1]
- Figure 2: Carbon emission by building type and Element[6]
- Figure 3: Opportunities to reduce embodied carbon across all project phases[9]
- Table 2: kg CO2e/m2 for traditional materials selected
- Table 3: kg CO2e/m2 for sustainable materials selected
- Figure 4: Different stages of the building renovation project[10]
- Figure 4: Types of the typical bays within the existing facade[10]
- Figure 5: Detail of typical bay(1A+1B)[10]